
Geology Final Water
Chapter 9:
P.264-284
Relative Dating Principles
Numerical vs Relative Dates
- Numerical dates specify the actual number of years that have passed since an event occurred, determined through radiometric dating techniques.1
- Relative dates establish the sequence of events (which came first, second, third, etc.) without providing numerical ages. Relative dating principles were developed before radiometric dating methods.1
Principle of Superposition
- In an undeformed sequence of sedimentary rocks, each bed is older than the one above and younger than the one below.1
Principle of Original Horizontality
- Sedimentary layers are generally deposited in a horizontal position. If layers are tilted or folded, it indicates they were deformed after deposition.1
Principle of Lateral Continuity
- Sedimentary beds originate as continuous layers that extend in all directions until they thin out at the edges of the depositional basin or grade into a different sediment type.1
Principle of Cross-Cutting Relationships
- Geologic features that cut across rocks (e.g. faults, igneous intrusions) must form after the rocks they cut through.1
Principle of Inclusions
- The rock mass containing inclusions (e.g. xenoliths) is younger than the inclusions themselves, which must have been incorporated from an older rock mass.1
Unconformities
- An unconformity represents a gap or missing interval in the rock record where deposition was interrupted by erosion or non-deposition.1
- Three types:
- Angular unconformity - Tilted older rocks overlain by younger flat-lying rocks
- Disconformity - Parallel layers with a gap representing a period of erosion
- Nonconformity - Sedimentary rocks over metamorphic/igneous rocks
Applying Relative Dating
- The principles allow geologists to determine the relative age sequence of rock units and geologic events represented, even without numerical dates.
- This establishes a basic geologic timeline for an area.
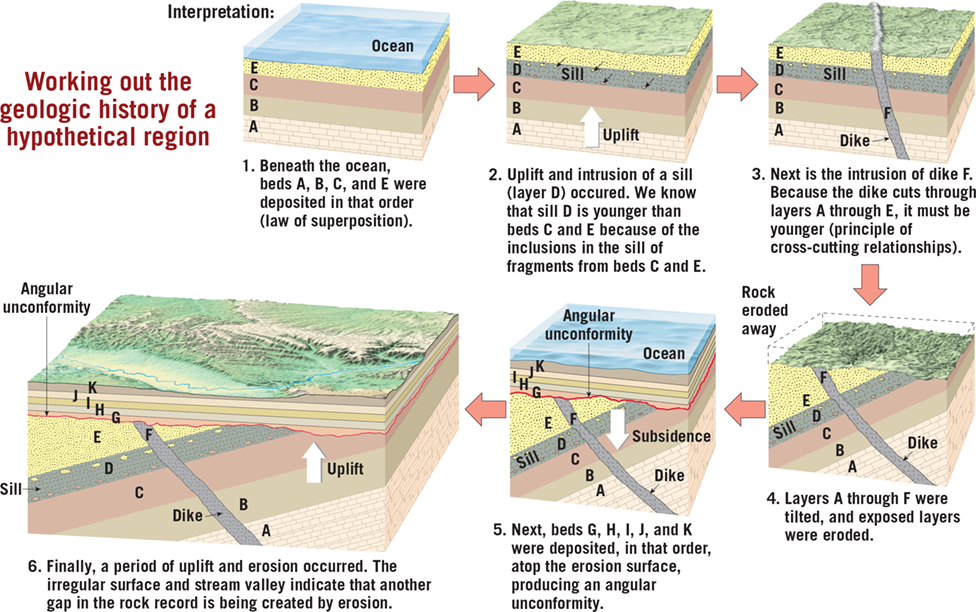
Review 9.1: p.271
1. Distinguish between numerical and relative dates.
- Answer: Numerical dates are specific calendar dates, while relative dates are based on the sequence of events without specific calendar dates.
2. Sketch and label four simple diagrams that illustrate each of the following: superposition, original horizontality, lateral continuity, and cross-cutting relationships.
- Answer:
- Superposition: Layers of rock are deposited on top of each other, with the oldest at the bottom and the youngest at the top.
- Answer:
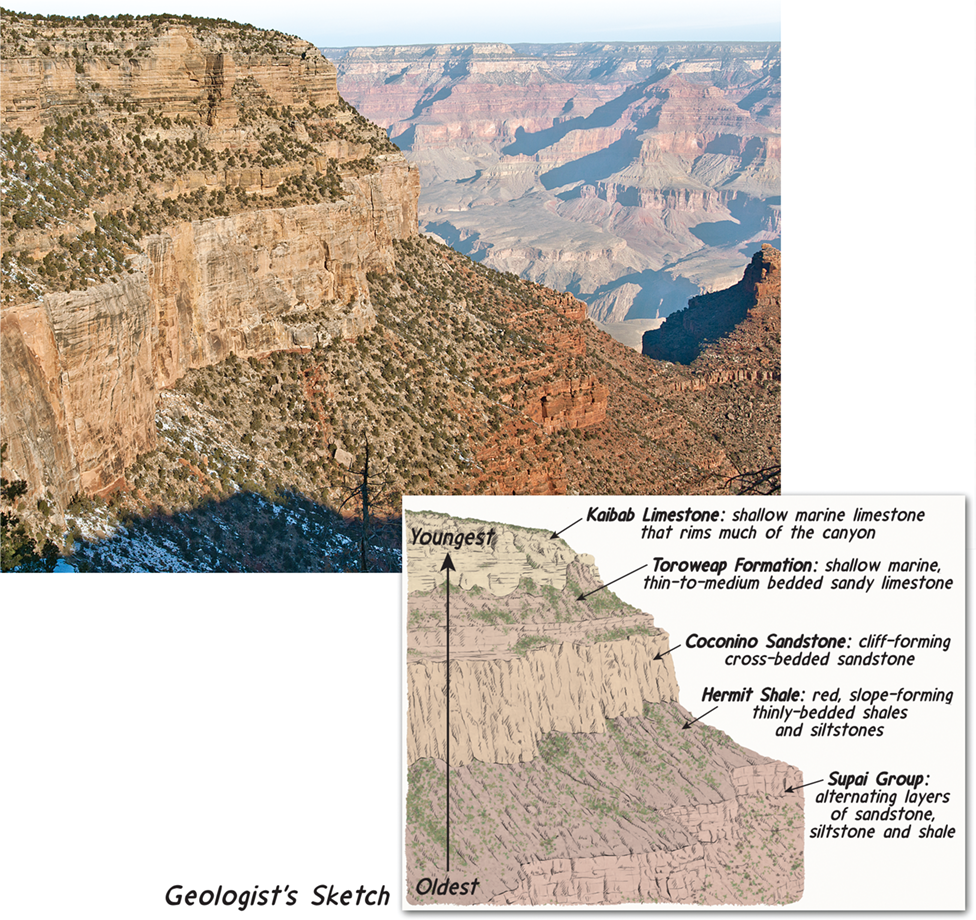
- Original horizontality: Sedimentary layers are initially deposited horizontally.
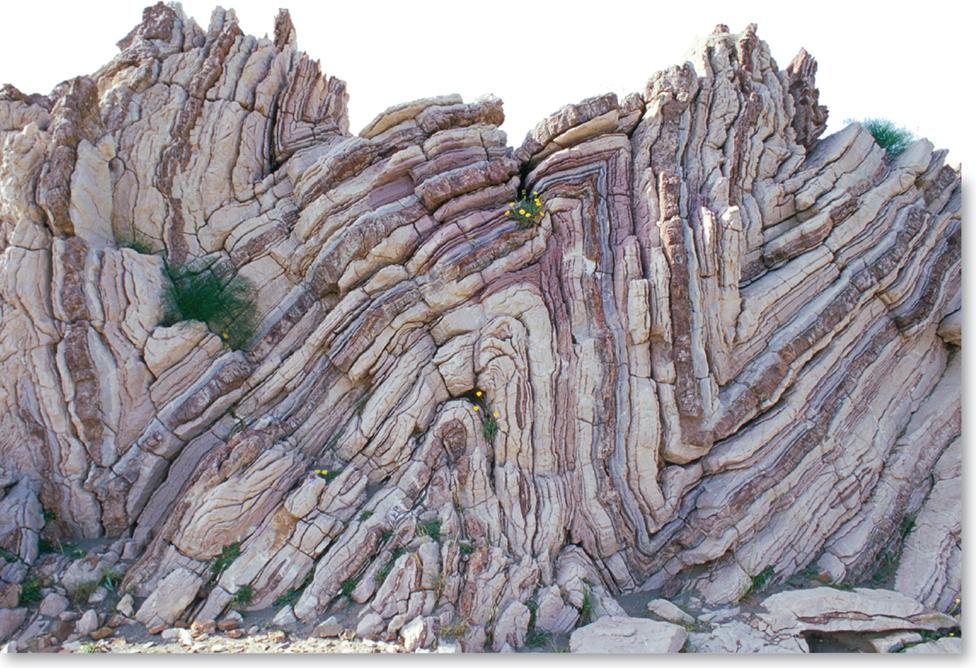
- Lateral continuity: Layers of sedimentary rock extend laterally in all directions until they thin out or grade into a different sediment type.
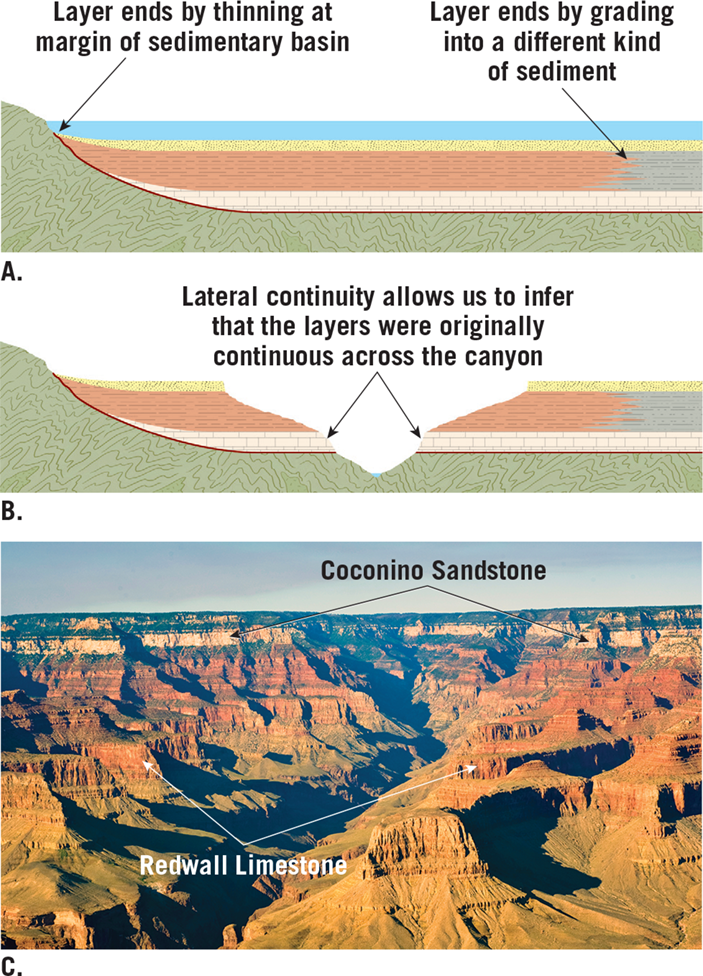
- Cross-cutting relationships: Any feature that cuts across or disrupts another rock layer or feature is younger than the one it cuts across.
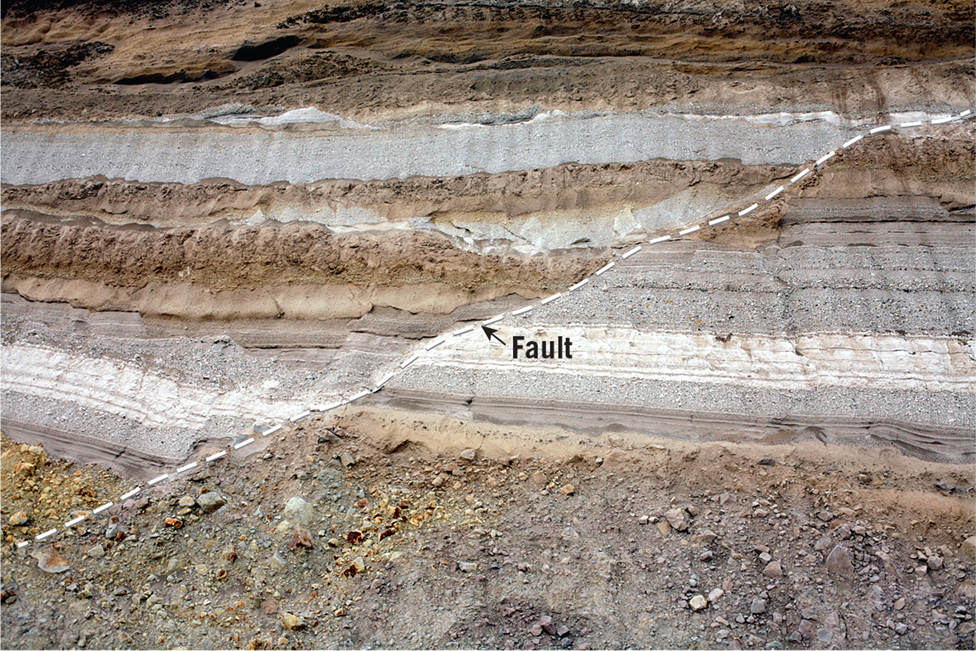
3. What is the significance of an unconformity?
- Answer: An unconformity represents a gap in the geologic record, indicating a period of erosion or non-deposition.
4. Sketch and explain the difference between an angular unconformity and a nonconformity.
- Answer:
- Angular unconformity: Layers of rock are tilted or folded, and then eroded before new sedimentary layers are deposited horizontally on top.
- Answer:
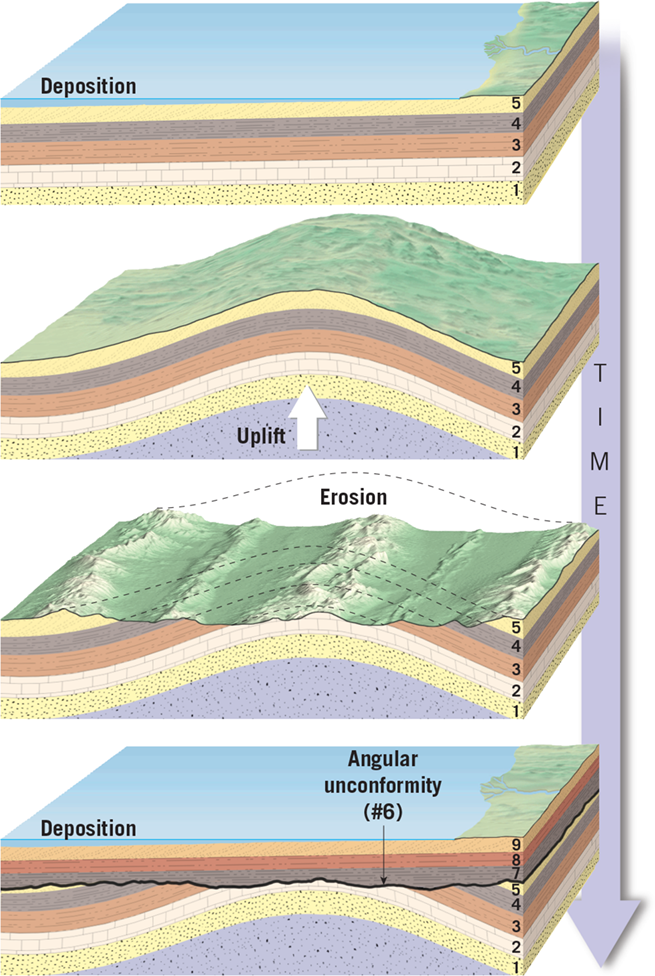
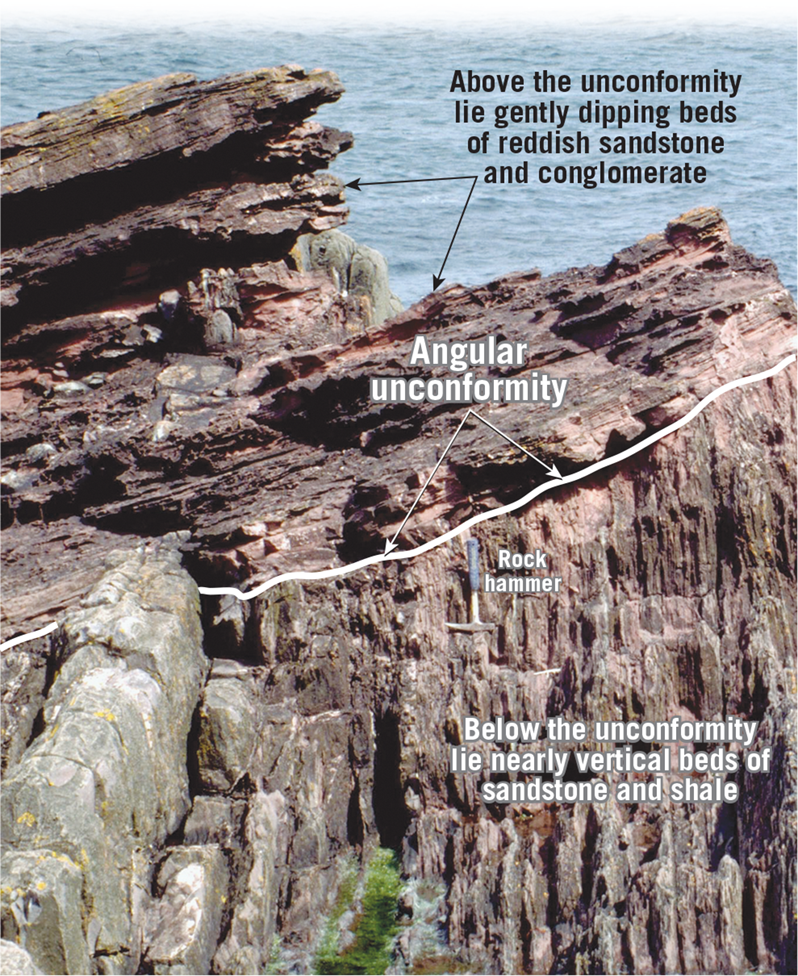
- Nonconformity: Sedimentary rocks overlie igneous or metamorphic rocks, indicating that the sedimentary layers were deposited on top of pre-existing, eroded rocks of a different type.
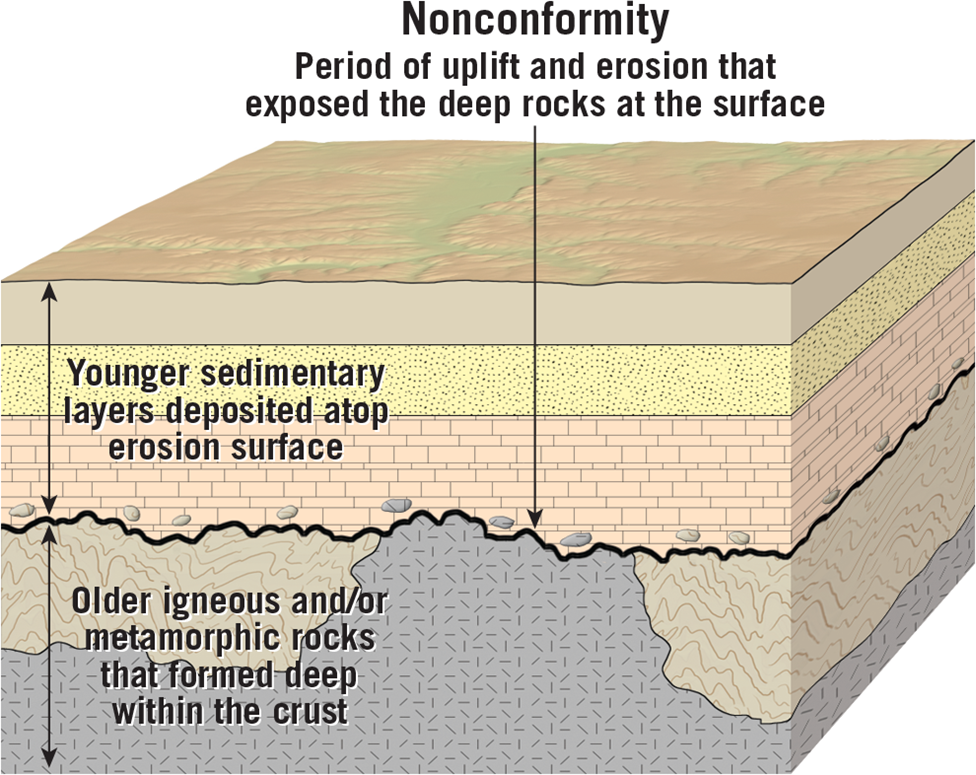
9.2 p.272 Fossils Evidence of Past Life
What is a Fossil?
A fossil is the preserved remains or traces of prehistoric life. Fossils form under specific conditions that allow the remains of organisms to become preserved in sedimentary rock layers.
Conditions Favoring Fossil Preservation
For an organism to become fossilized, two main conditions are required:
- Rapid burial in sediment after death - This protects the remains from destructive surface processes.
- Possession of hard parts (bones, shells, teeth, woody stems) - Soft tissues decay quickly before they can be preserved.
Less than 10% of all organisms that ever existed are represented in the fossil record due to the specific conditions required for fossilization.
Types of Fossils
- Body Fossils
- Preserved actual remains like bones, teeth, shells
- Permineralization - porous tissues filled by mineral deposits
- Petrified wood - permineralized wood turned to stone
- Molds and casts - hollow molds or mineral-filled casts of organisms
- Trace Fossils
- Indirect evidence of life like footprints, burrows, coprolites (fossil dung)
- Carbonization and Impressions
- Carbon films or impressions left in fine sediment by delicate organisms
- Amber
- Organisms trapped and preserved in ancient tree resin
The fossil record is biased towards organisms with hard parts that lived in areas conducive to sedimentation and burial. Soft-bodied organisms are rarely preserved as fossils.13
Fossils provide evidence of the progression and evolution of life over millions of years by showing changes in complexity, diversity, and the appearance and extinction of different life forms through geologic time.13 The fossil record is crucial for understanding prehistoric environments and life on Earth.
9.2 Review
There are several ways an organism can be preserved as a fossil:
- Permineralization - This occurs when mineral-rich groundwater permeates the porous tissues like bone or wood, and minerals precipitate out filling the empty spaces. This turns the organism into a rock-like fossil, such as petrified wood.
- Molds and casts - If a shell or body imprint is left in sediment, it creates a mold. If this hollow mold later gets filled with minerals, it creates a cast fossil which is a replica of the original organism.
- Carbonization - This preserves delicate organisms like leaves or feathers by the remains being encased in fine sediment, squeezed by pressure, leaving just a thin carbon film outline.
- Amber - Some organisms like insects can get trapped and preserved in ancient tree resin that hardens into amber, protecting them from decay.13
Question: List two examples of trace fossils.
Two examples of trace fossils are:
- Tracks/Footprints - Impressions left by an animal's footprints in soft sediment that later hardens into rock.
- Burrows - Tunnels or holes dug into sediment or wood by an animal, that later get filled in by mineral deposits.13
Question: What conditions favor the preservation of an organism as a fossil?
The key conditions that favor an organism being preserved as a fossil are:
- Rapid burial in sediment after death - This protects the remains from destructive surface processes and scavengers.
- Possession of hard parts like shells, bones, teeth - Soft tissues decay too quickly to fossilize easily.
- Anaerobic (oxygen-poor) conditions - Limits bacterial decay and decomposition of the remains.
- Cold, dry conditions - Such as Arctic permafrost, which can preserve remains like woolly mammoths for thousands of years.1245
So in summary, the ideal conditions are rapid burial in an anaerobic, mineral-rich sediment that allows permineralization, in a cold, dry environment that limits decomposition - especially for organisms with hard body parts.
9.3 p.274-275
Correlating Rock Layers Using Fossils
Principle of Fossil Succession
- Fossil organisms succeed each other in a definite and determinable order through geologic time
- Any time period can be recognized by its characteristic fossil content
- This succession of life forms is found in the same order on every continent
Index Fossils
- Widespread geographically but limited to a short span of geologic time
- Presence of an index fossil allows matching of rock layers of the same age
Fossil Assemblages
- If no index fossil is present, a group of fossils (assemblage) can be used to date a rock layer
- Overlapping ranges of multiple fossil species allows more precise dating
Using Fossils as Environmental Indicators
Interpreting Depositional Environments
- Fossil evidence helps deduce the ancient environment the sediments were deposited in
- E.g. Clam fossils in limestone = shallow marine environment
Identifying Shoreline Position
- Thick-shelled fossils = high energy shoreline environment
- Thin, delicate shells = deeper, calmer offshore waters
Determining Water Temperature
- Fossil coral = ancient warm, shallow tropical seas
- Allows interpretation of past climate and water temperatures
In summary, fossils are crucial for correlating rock layers between locations and reconstructing the environmental conditions, climate, and geography that existed when those rocks formed millions of years ago.
9.3 Review p.275
Question: What is the goal of correlation?
The goal of correlation is to match up rock layers of the same age across different geographic areas, even when they are composed of different lithologies (rock types). By correlating rocks from separate locations, geologists can piece together a more comprehensive picture and understanding of Earth's geologic history over a wider region.14
Question: State the principle of fossil succession in your own words.
The principle of fossil succession states that fossil organisms are preserved in sedimentary rock layers in a specific and consistent order that represents their relative timing and evolution through geologic time. Older, more primitive fossil forms will be found in lower rock layers, while younger and more modern fossil forms will be found in higher overlying layers. This ordered succession of fossil types is found globally.135
Question: Along with being important in correlation, how else are fossils useful to geologists?
In addition to their crucial role in correlating rock layers, fossils are also useful to geologists as:
- Environmental indicators - Fossils provide evidence about the ancient environments the sediments were deposited in, such as marine vs terrestrial, shallow vs deep water, warm vs cold climates, etc.13
- Dating rocks - The presence of certain index fossils with short temporal ranges can be used to relatively date when that rock layer was formed.34
- Interpreting evolution - The succession of fossil forms from older to newer shows the pattern and progression of evolution over time.5
- Reconstructing geography - Fossils indicate positions of ancient shorelines, depths of water bodies, locations of landmasses, etc. when combined with other sedimentary evidence.1
So in summary, while critical for correlation, fossils are also invaluable tools that allow geologists to decipher ancient environments, date rocks, trace evolution, and reconstruct the paleogeography of our planet through deep time.
9.4 Numerical Dating with Nuclear Decay
Three Types of Radioactive Decay
- Alpha Decay:
- Definition: Emission of alpha particles (2 protons and 2 neutrons) from the nucleus.
- Effect: Mass number decreases by 4, atomic number decreases by 2.
- Beta Decay:
- Definition: Emission of an electron from the nucleus, converting a neutron to a proton.
- Effect: Mass number remains unchanged, atomic number increases by 1.
- Electron Capture:
- Definition: Capture of an electron by the nucleus, converting a proton to a neutron.
- Effect: Mass number remains unchanged, atomic number decreases by 1.
Use of Radioactive Isotopes in Numerical Dating
- Unstable isotopes (parents) decay into stable isotopes (daughters) at a steady rate.
- By measuring the ratios of parent to daughter isotopes in a sample, the age of the sample can be calculated.
Radiometric Dating
- Half-Life: Time required for half of the nuclei in an unstable isotope to decay.
- Calculation: Age of a sample = (Number of half-lives elapsed) × (Half-life of the isotope).
Unstable Isotopes Used for Radiometric Dating
- Useful isotopes include Rubidium-87, Thorium-232, Uranium-235, Uranium-238, and Potassium-40.
- Potassium-40 is versatile and used for dating rocks younger than 100,000 years.
Sources of Error in Radiometric Dating
- Leakage of parent or daughter isotopes.
- Thermal resetting, especially in the case of Argon-40.
- Weathering or leaching, causing loss of daughter atoms.
Earth's Oldest Rocks
- Rocks exceeding 3.5 billion years in age found globally.
- Radiometric dating confirms immense geologic time.
Dating with Carbon-14
- Radiocarbon Dating: Uses carbon-14 to date organic materials.
- Effective for dating events from the historic past up to 70,000 years ago.
- Carbon-14 is continuously produced in the atmosphere and incorporated into living organisms.
Importance of Carbon-14 Dating
- Valuable for anthropologists, archaeologists, historians, and geologists studying recent Earth history.
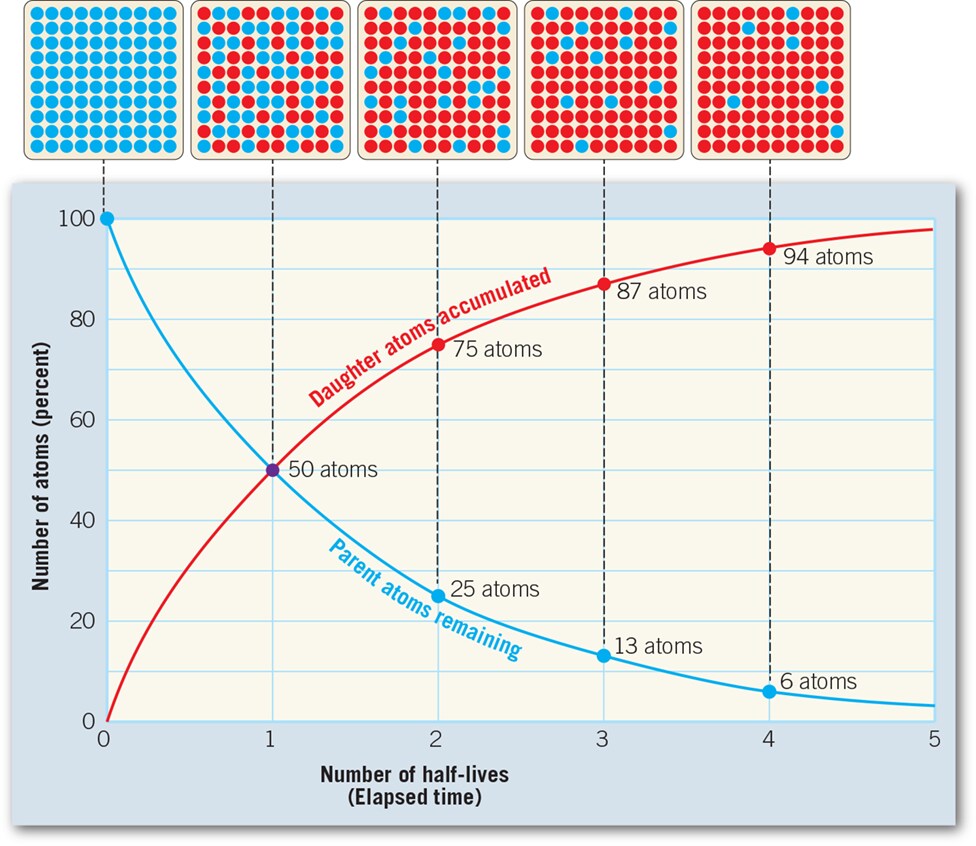
Review 9.4
- List three types of radioactive decay. For each type, describe how the atomic number and atomic mass change.
- Alpha Decay:
- Change: Atomic mass decreases by 4, atomic number decreases by 2.
- Beta Decay:
- Change: Atomic mass remains unchanged, atomic number increases by 1.
- Electron Capture:
- Change: Atomic mass remains unchanged, atomic number decreases by 1.
- Alpha Decay:
- Sketch a simple diagram to explain the idea of half-life.
- [Sketch of a graph with time on the x-axis and remaining radioactive material on the y-axis. The graph shows a steady decrease in the amount of radioactive material over time, with each half-life representing a halving of the remaining material.]
- For what time span does radiocarbon dating apply?
- Answer: Radiocarbon dating can be used to date events from the historic past as well as those from very recent geologic history, typically up to around 70,000 years ago.
9.5 Determining Numerical Dates for Sedimentary Strata
Reliability of Numerical Dates for Sedimentary Rocks
- Explanation: Determining reliable numerical dates for layers of sedimentary rock poses challenges due to the inability to directly date sedimentary rocks using radiometric methods.
Radiometric Dating Limitations for Sedimentary Rocks
- Explanation: Sedimentary rocks rarely yield accurate radiometric ages because their grains are not the same age as the rock itself, having been weathered and eroded from rocks of diverse ages. Similarly, radiometric dates from metamorphic rocks may not represent the rock's initial formation but rather subsequent metamorphic phases.
Relationship to Igneous Rocks
- Explanation: Numerical dates for sedimentary layers are often determined by their relationship to datable igneous rocks. Geologists relate sedimentary strata to nearby igneous masses to establish numerical dates.
Example: Dating Sedimentary Strata
- Explanation: Radiometric dating of volcanic ash beds and intruding dikes provides numerical dates for sedimentary layers. Layers above the ash are younger, while those below are older. Dikes are younger than the rocks they cut through but older than the rocks they intrude.
Combining Methods for Dating
- Explanation: Geologists combine laboratory dating methods with relative dating principles to estimate the ages of sedimentary strata. This involves examining relationships between sedimentary layers and datable igneous rocks to bracket specific time periods in Earth's history.
Conclusion:
Determining numerical dates for sedimentary strata involves overcoming the limitations of radiometric dating by relying on relationships with igneous rocks. By combining laboratory dating techniques with field observations, geologists can estimate the ages of sedimentary layers and gain insights into Earth's history.
9.5 Review p. 282
- Briefly explain why it is often difficult to assign a reliable numerical date to a sample of sedimentary rock.
- Answer: Assigning a reliable numerical date to sedimentary rock samples is challenging because sedimentary rocks rarely contain minerals that can be directly dated using radiometric methods. The grains within sedimentary rocks are typically not the same age as the rock itself, having been weathered and eroded from various sources, making direct dating unreliable.
- How might a numerical date for a layer of sedimentary rock be determined?
- Answer: Numerical dates for sedimentary rock layers are often determined by examining their relationship to nearby igneous rocks. Geologists utilize radiometric dating techniques on igneous rocks that intrude or overlie sedimentary layers. By dating volcanic ash beds or igneous dikes within or adjacent to sedimentary layers, geologists can establish numerical ages for those sedimentary strata based on their relative positions to the dated igneous rocks.
9.6 The Geologic Time Scale
Structure of the Geologic Time Scale
- Explanation: The geologic time scale divides Earth's 4.6-billion-year history into various units, with eons representing the broadest expanses of time, followed by eras, periods, and epochs.
Basic Time Units:
- Eons:
- Definition: The broadest divisions of time, with the Phanerozoic eon (541 million years ago to present) containing abundant fossil evidence.
- Eras:
- Definition: Subdivisions of eons, including the Paleozoic, Mesozoic, and Cenozoic eras, characterized by significant changes in life-forms.
- Periods:
- Definition: Subdivisions of eras, such as the Paleozoic with seven periods, representing more specific changes in life evolution.
- Epochs:
- Definition: Smaller units within periods, providing finer detail of geological time, particularly in the Cenozoic era.
Precambrian Time
- Explanation: Represents the vast expanse of Earth's history before the Cambrian period, comprising the Archean and Proterozoic eons.
- Challenges: Difficult to subdivide due to lack of detailed knowledge and the absence of abundant fossil evidence.
Dynamic Nature of the Time Scale
- Explanation: The geologic time scale is continually refined as new evidence and understanding of Earth's history emerge.
- Updates: The International Commission on Stratigraphy periodically updates the time scale to include changes in unit names and boundary age estimates.
Informal Terms
- Explanation: Terms like "Precambrian" and "Hadean" are informally used despite not being officially recognized on the geologic time scale.
- Purpose: Aid effective communication in the geosciences despite lacking formal status.
Anthropocene
- Explanation: Some scientists propose the Anthropocene as a new epoch, reflecting human-caused global environmental changes.
- Debate: There's ongoing debate about when the Anthropocene began and whether it should be officially recognized as a geologic epoch.
- Dynamic Tool: Recognizes the evolving nature of Earth's history and our understanding of it.
Conclusion:
The geologic time scale provides a framework for understanding Earth's complex history, with divisions ranging from eons to epochs. It evolves as our knowledge advances, reflecting the dynamic nature of Earth's geological processes and the impact of human activities.
9.6 Review p.
- List the four basic units that make up the geologic time scale.
- Answer: The four basic units of the geologic time scale are eons, eras, periods, and epochs.
- What term applies to all of geologic time prior to the Phanerozoic eon? Why is this span not divided into epochs as is the Phanerozoic eon?
- Answer: The term "Precambrian" applies to all of geologic time prior to the Phanerozoic eon. This span is not divided into epochs like the Phanerozoic eon because the Precambrian lacks detailed knowledge and abundant fossil evidence. The rocks from this time are highly distorted and metamorphosed, making interpretation difficult.
- Explain why scientists occasionally change the geologic time scale.
- Answer: Scientists occasionally change the geologic time scale to incorporate new discoveries, refine dating techniques, and update unit names and boundary age estimates. This ensures that the time scale remains accurate and reflects the latest understanding of Earth's history.
Question:
- The accompanying diagram is a cross section of a hypothetical area. Place the lettered features in the proper sequence, from oldest to youngest. Where in the sequence can you identify an unconformity? Which principles did you use to establish the sequence?
Question
- Measurements of zircon crystals containing trace amounts of uranium from a specimen of granite yield parent/daughter ratios of 25 percent parent (uranium-235) and 75 percent daughter (lead-206). The half-life of uranium-235 is 704 million years. How old is the granite?
Question
Express the numerical age of the sandstone layer in the diagram as accurately as possible.
Chapter 16
The Hydrologic Cycle
Earth's Water Reservoirs
- The vast majority (96.5%) of Earth's water is stored in the global oceans145.
- The remaining freshwater is found in glaciers and ice sheets (1.7%), groundwater (1.7%), lakes and rivers (0.01%), and the atmosphere (0.001%)145.
Paths of Water in the Hydrologic Cycle
- The hydrologic cycle is powered by energy from the Sun and involves the continuous circulation of water through Earth's land, oceans, and atmosphere12345.
- Water enters the atmosphere primarily through evaporation from the oceans, with a smaller contribution from transpiration by plants1245.
- Winds transport water vapor, which condenses to form clouds and eventually precipitates back to the Earth's surface12345.
- Precipitation that falls on land can either infiltrate the soil and become groundwater, run off into streams and rivers, or evaporate back into the atmosphere12345.
- Groundwater can slowly seep into lakes, streams, and the ocean, completing the cycle12345.
Water Balance
- The total amount of water vapor in the atmosphere remains relatively constant, so the average annual precipitation worldwide must equal the amount of water evaporated145.
- However, precipitation exceeds evaporation over land, while evaporation exceeds precipitation over the oceans145.
- This imbalance is resolved by the vast amount of water (36,000 cubic km annually) that flows from land back to the ocean145.
Importance of the Hydrologic Cycle
- The hydrologic cycle is a crucial process that shapes Earth's land surface through erosion, flooding, and groundwater formation145.
- It also provides the water resources necessary to sustain life and human activities on land1245.
- Evaporation: Liquid water changes into water vapor and enters the atmosphere from oceans and land.
- Transpiration: Plants release water vapor into the atmosphere.
- Precipitation: Water vapor condenses and falls back to Earth's surface as rain, snow, sleet, or hail.
- Infiltration: Precipitation that falls on land may soak into the ground, moving laterally and eventually seeping into lakes, streams, or the ocean.
- Runoff: Excess water flows over the surface into lakes and streams when infiltration capacity is exceeded.
- Storage in Glaciers: Precipitation in cold regions accumulates as snow or ice, forming glaciers that store large quantities of water on land.
- Evapotranspiration: Combined process of evaporation from soil, lakes, and streams, along with transpiration from plants, transferring water back to the atmosphere.
16.1 Review
- Describe or sketch the movement of water through the hydrologic cycle. Once precipitation has fallen on land, what paths might the water take?
- In the hydrologic cycle, water moves through various stages:
- Evaporation: Liquid water from oceans and land surfaces turns into water vapor and rises into the atmosphere.
- Transpiration: Plants release water vapor through their leaves.
- Condensation: Water vapor condenses to form clouds.
- Precipitation: Condensed water falls back to Earth's surface as rain, snow, sleet, or hail.
- Infiltration: Precipitation that lands on the ground may seep into the soil.
- Runoff: Excess water flows over the surface into rivers, lakes, and oceans.
- Storage in Glaciers: Some precipitation accumulates as snow and forms glaciers, storing water on land.
- Evapotranspiration: Combined process of evaporation from soil, lakes, and streams, along with transpiration from plants, returns water vapor to the atmosphere.
- In the hydrologic cycle, water moves through various stages:
- Once precipitation has fallen on land, water might follow various paths:
- Soak into the ground (infiltration)
- Flow over the surface (runoff)
- Be absorbed by plants and later released back into the atmosphere (transpiration)
- Accumulate in rivers, lakes, or groundwater reservoirs
- Eventually return to the ocean through runoff or groundwater flow.
- What is meant by the term evapotranspiration?
- Evapotranspiration refers to the combined process of evaporation and transpiration. It involves the transfer of water from the Earth's surface, such as soil, lakes, and streams, into the atmosphere. Evaporation occurs when liquid water turns into water vapor from surfaces like soil and bodies of water. Transpiration occurs when plants absorb water through their roots and release water vapor through their leaves into the atmosphere. Together, evaporation and transpiration contribute to the water cycle by returning water vapor to the atmosphere.
- Over the oceans, evaporation exceeds precipitation, yet sea level does not drop. Explain why.
- Although evaporation exceeds precipitation over the oceans, sea level does not drop because water is constantly cycled back to the oceans through other pathways. The excess water evaporated from the oceans is replenished by precipitation falling on land. This water eventually makes its way back to the oceans through runoff, groundwater flow, and rivers. Additionally, melting ice from glaciers and polar ice caps also contributes to maintaining sea levels. Overall, the hydrologic cycle ensures that water is continuously redistributed, preventing a significant drop in sea level despite regional differences in evaporation and precipitation.
The hydrologic cycle -- The Earth's water transfer system is called the hydrologic cycle. The water system is powered by the sun. 97.2% of the Earth's total water is in the oceans. 2.2% is in ice caps and glaciers. 0.6% is in groundwater, rivers, lakes and the atmosphere.
Freshwater budget -- Groundwater is 14% of freshwater in hydrosphere. The average rate of groundwater exchange is 280 years. Exchange means the transfer from the atmosphere to the ground and back to the atmosphere. This exchange rate gives an indication that groundwater movement is slow.
Water table -- The water table defines the top of the zone of saturation, where groundwater occurs. Porosity governs the amount of groundwater that a rock material can hold. The water table is not flat. The water table is higher under hills than under valleys. Groundwater flow is impeded by indirect flow around grains.
Permeability -- Permeability defines the ability of a porous rock to transmit a fluid. Permeable layers are called aquifers. Impermeable layers are called aquicludes. Springs arise from a perched water table. Otherwise groundwater discharges into streams as a consequence of flowing from a higher water under hills towards the lower water table in valleys. If water discharges into a stream, the water table comes to surface right at the stream. In this case the water table slopes downhill toward the stream.
Mining Groundwater -- Wells are drilled into the zone of saturation below the water table. When wells are pumped, the water flows to the well which locally depresses the water table (drawdown) and creates a cone of depression.
Groundwater is an exhaustible resource.
River Systems (Section 16.2)
1. Nature of Drainage Basins and River Systems:
- Drainage Basin Definition: An area of land drained by a river and its tributaries.
- River System Definition: Includes the network of stream channels and the entire drainage basin.
- Key Concepts:
- Drainage basin bounded by a divide, with an outlet at a lower elevation.
- Drainage divides can vary in scale from small ridges to continental divides.
- Example: The Mississippi River drainage basin is the largest in North America.
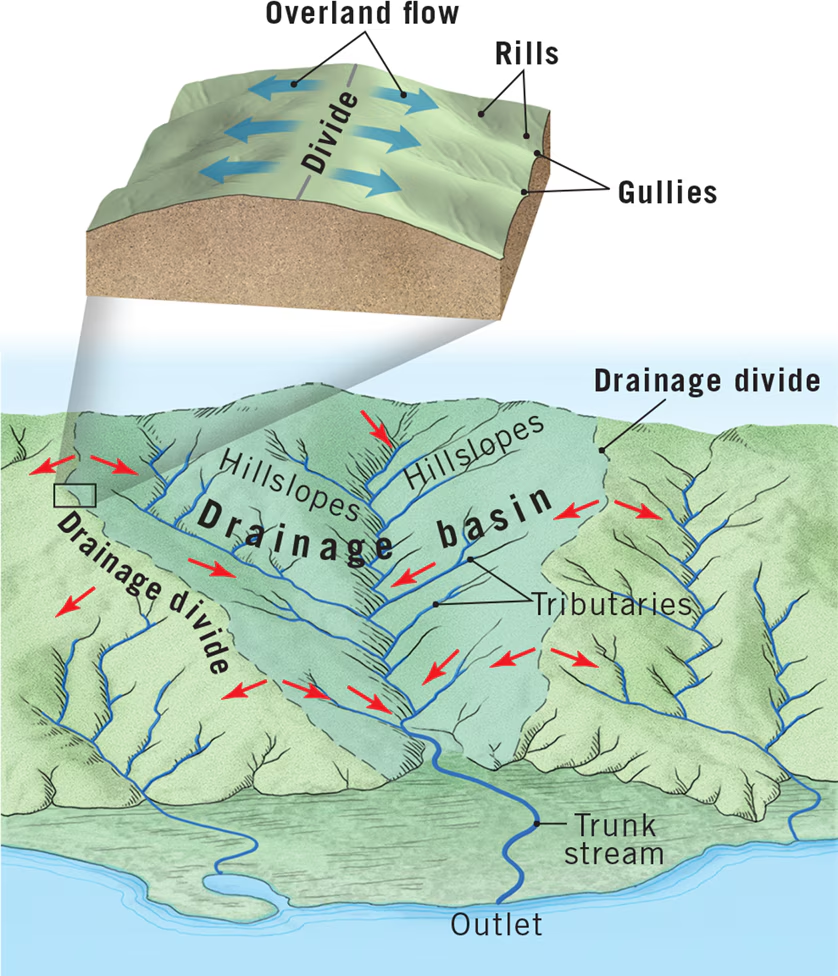
2. Factors Influencing Runoff:
- Factors:
- Intensity and duration of rainfall.
- Soil moisture content.
- Surface material permeability.
- Slope of the land.
- Vegetation type and extent.
- Runoff Dominance: Occurs when surface material is impermeable or saturated, leading to high runoff.
3. Processes of Stream Formation:
- Sheet Flow: Initial runoff across slopes.
- Rills: Tiny channels formed from sheet flow.
- Gullies: Channels formed by merging rills.
- Streams and Rivers: Formed by merging gullies and tributaries.
4. Sources of Streamflow:
- Humid Regions: Streamflow from overland flow and groundwater.
- Arid Regions: Stream loses water to groundwater due to lower water table level.
5. Drainage Patterns:
- Dendritic Pattern: Irregular tributary streams resembling tree branches. Most common. Because the surface material is essentially uniform in its resistance to erosion, it does not control the pattern of streamflow. Rather, the pattern is determined chiefly by the direction of slope of the land.
- Radial Pattern: Streams diverge from a central area like spokes on a bike tire, common in volcanic regions.
- Rectangular Pattern: Streams with right-angle bends due to underlying structural features.
- Trellis Pattern: Parallel tributary streams, common in areas with alternating bands of resistant rock.
6. River Zones and Sediment Dynamics:
- Zones: Sediment production, transport, and deposition.
- Sediment Production: Occurs in headwaters through weathering and erosion.
- Sediment Transport: Sediment moves through trunk streams.
- Sediment Deposition: Occurs at river mouths, forming deltas or coastal features.
7. River Evolution:
- Headward Erosion: Lengthening of streams by erosion of their heads upslope.
- Antecedent Streams: Streams that maintain their course despite uplift.
- Superposed Streams: Streams that erode through existing structures due to sedimentary layering.
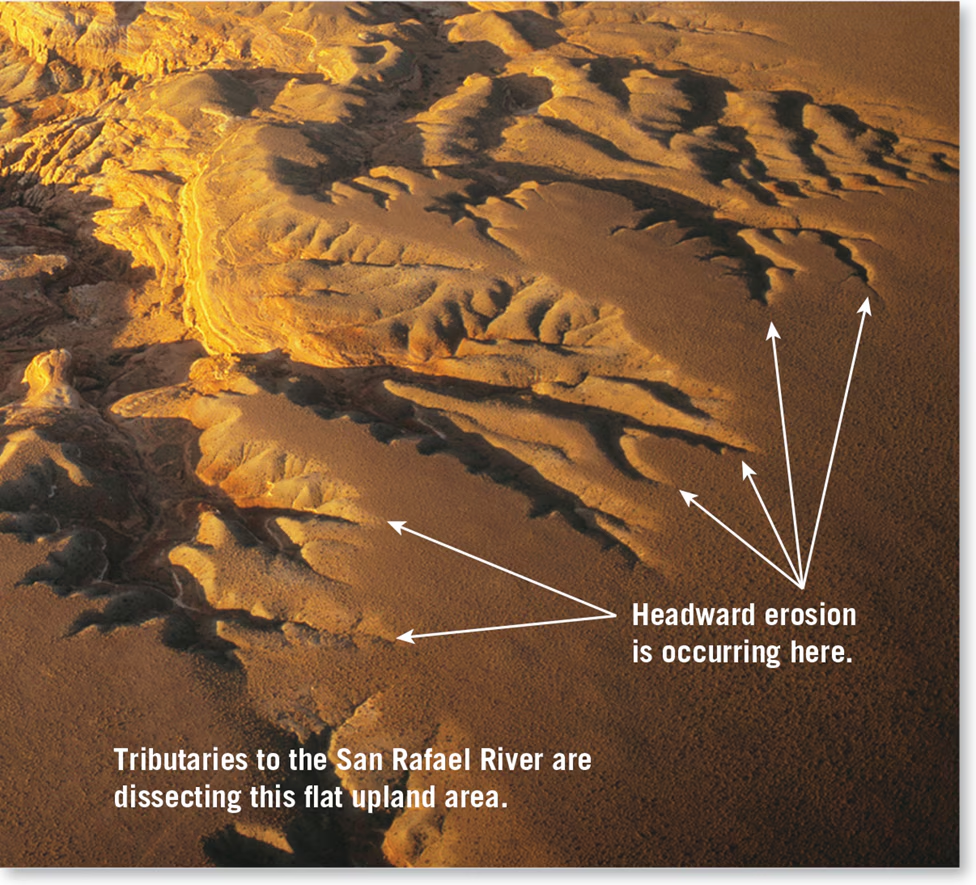
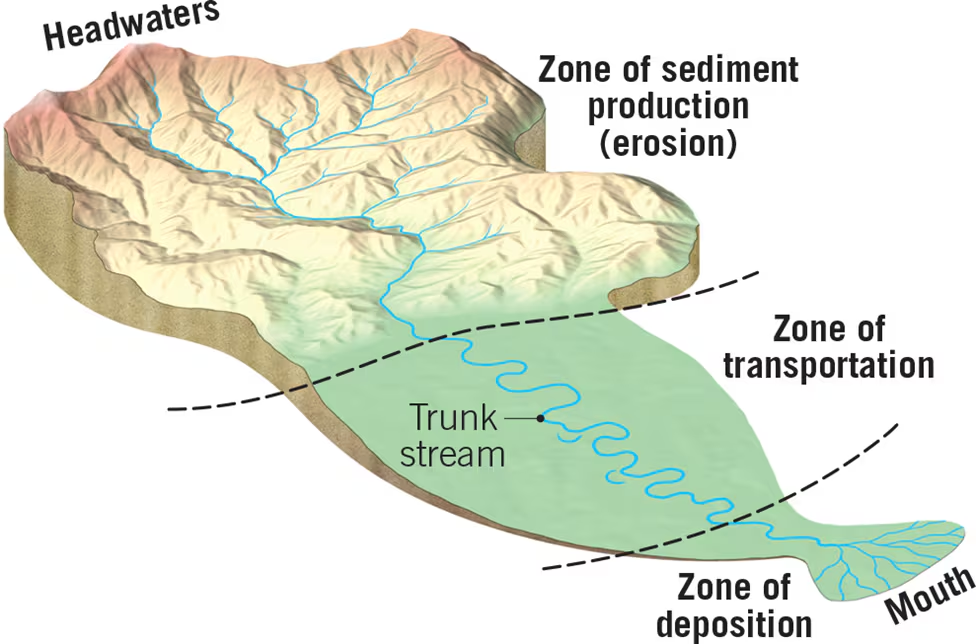
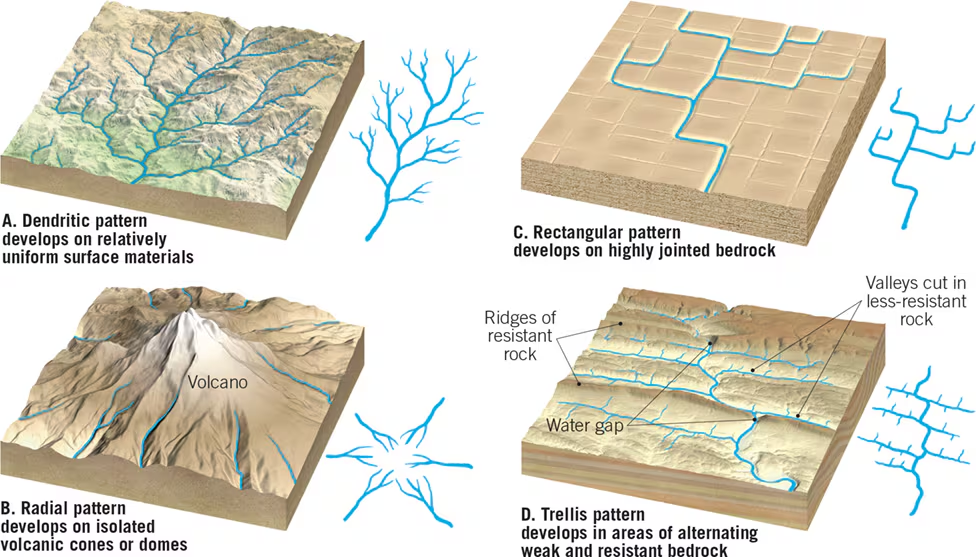
Summary: 16.2
Understanding river systems involves grasping concepts such as drainage basins, runoff factors, stream formation processes, sources of streamflow, drainage patterns, sediment dynamics, and river evolution. Each aspect contributes to the complex behavior and morphology of rivers, shaping landscapes over time.
16.2 Review Questions
Factors influencing infiltration and runoff variability:
- Rainfall Intensity and Duration: Heavy rainfall over a short period increases runoff, while light rainfall over a longer duration promotes infiltration.
- Soil Type and Moisture Content: Sandy soils facilitate infiltration, while clayey soils can lead to surface runoff.
- Topography: Steeper slopes enhance runoff due to gravity, while flat areas allow more time for infiltration.
- Vegetation Cover: Dense vegetation slows down runoff by intercepting rainfall and promoting infiltration through root systems.
- Human Activities: Urbanization, deforestation, and land use changes can increase surface runoff by creating impermeable surfaces.
Simple sketch of a drainage basin and a divide:
- Drainage Basin: Area drained by a river and its tributaries.
- Divide: Imaginary line separating two adjacent drainage basins.
Three main parts (zones) of a river system:
- Zone of Sediment Production: Located in the headwaters region where erosion dominates, contributing sediment to the river.
- Zone of Sediment Transport: The main channel network where sediment is transported downstream by the river.
- Zone of Sediment Deposition: Where the river reaches a body of water, slowing down and depositing sediment, forming deltas or coastal features.
Brief description of the four main stream patterns and their causes:
- Dendritic Pattern: Irregular tributary streams resembling tree branches. Occurs in areas with uniform underlying geology and slope.
- Radial Pattern: Streams diverge from a central area like spokes from a wheel hub. Common in volcanic regions.
- Rectangular Pattern: Streams with right-angle bends due to underlying structural features like joints or faults.
- Trellis Pattern: Parallel tributary streams resembling a garden trellis. Forms in areas with alternating bands of resistant and less-resistant rock, often seen in folded mountain ranges.
16.3 Streamflow Characteristics
1. Streamflow and its Characteristics:
- Definition: Streamflow refers to the movement of water within river channels under the influence of gravity. It encompasses the various behaviors and properties of water as it moves through channels, including velocity, turbulence, and erosion capacity.
- Flow Types:
- Laminar Flow: This type of flow occurs when water moves smoothly in parallel layers, with minimal mixing between adjacent layers. It is characterized by streamlined paths and low turbulence. Laminar flow is typically observed in very slow-moving streams and is governed by viscosity and inertia.
- Turbulent Flow: Turbulent flow is characterized by irregular and chaotic movement of water, with mixing occurring between adjacent layers. It is commonly observed in natural streams and rivers, especially in areas with high velocities or obstacles. Turbulence contributes to increased erosion and sediment transport capacity.
2. Factors Influencing Streamflow:
- Flow Velocity: Flow velocity refers to the speed at which water moves within a stream channel. It is influenced by various factors including channel gradient, shape, size, roughness, and discharge. Higher velocities are associated with increased turbulence and erosion potential.
- Gradient: Gradient, also known as slope, represents the change in elevation of a stream channel over a given distance. Steeper gradients result in higher flow velocities and increased erosional power, while gentler gradients lead to slower velocities and reduced erosional capacity.
- Channel Shape: The shape of the stream channel cross-section significantly impacts flow characteristics. Channel shape influences the wetted perimeter, which is the portion of the channel in contact with water. A smaller wetted perimeter reduces frictional resistance and promotes higher flow velocities.
- Channel Size and Roughness: Larger channels generally exhibit higher flow velocities compared to smaller channels due to increased cross-sectional area and discharge. Additionally, the roughness of the channel bed and banks affects flow velocity, with smoother surfaces reducing frictional resistance and enhancing flow.
- Discharge: Discharge refers to the volume of water passing a specific point in the stream channel over a given time period. It is calculated by multiplying the cross-sectional area of the channel by the flow velocity. Discharge is a key determinant of a stream's capacity for erosion, sediment transport, and hydraulic efficiency.
3. Monitoring Streamflow:
- The monitoring of streamflow involves the collection and analysis of data related to flow velocity, discharge, and river stage. This data is typically obtained through a network of stream gaging stations maintained by organizations such as the U.S. Geological Survey (USGS).
- Streamflow data is essential for various purposes, including flood forecasting, water resource management, infrastructure design, and environmental monitoring. It provides valuable insights into the behavior and dynamics of river systems, aiding in decision-making and risk assessment.
4. Changes from Upstream to Downstream:
- Longitudinal Profile: The longitudinal profile of a stream represents a cross-sectional view of its channel from its source (headwaters) to its mouth (where it joins another body of water). It typically exhibits a gradual decrease in gradient from upstream to downstream.
- Gradient Decrease: As streams flow downstream, the slope of the channel, or gradient, generally decreases. This reduction in gradient is accompanied by an increase in discharge and channel size. While the decrease in gradient lowers the energy available for erosion, the larger channel dimensions and increased discharge compensate for this reduction, resulting in similar or higher flow velocities downstream.
- Sediment Changes: Along most rivers, changes in sediment characteristics occur from upstream to downstream. Sediment size tends to decrease downstream, leading to smoother channel beds and increased hydraulic efficiency. This downstream fining of sediment particles is a result of selective transport, with coarser particles settling out closer to the source while finer particles remain in suspension and travel further downstream.
Summary:
Understanding streamflow characteristics involves considering factors such as flow velocity, gradient, channel shape, size, roughness, discharge, and sediment dynamics. Monitoring streamflow through gaging stations provides valuable data for various applications, including flood forecasting and water resource management. Changes in stream characteristics from upstream to downstream, including gradient decrease and sediment changes, contribute to the overall behavior and morphology of river systems.
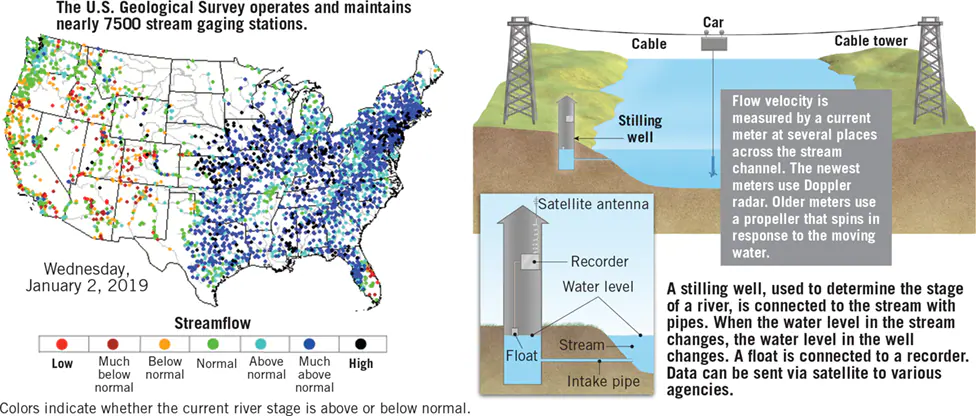
16.3 Review Questions
1. Contrast laminar flow and turbulent flow:
Laminar Flow:
- Description: Laminar flow occurs when water moves smoothly in parallel layers, with minimal mixing between adjacent layers.
- Characteristics:
- Smooth, streamlined paths.
- Low turbulence.
- Predominant in slow-moving streams.
- Factors: Governed by viscosity and inertia.
- Effect: Minimal mixing between layers, resulting in uniform flow.
Turbulent Flow:
- Description: Turbulent flow is characterized by irregular and chaotic movement of water, with mixing occurring between adjacent layers.
- Characteristics:
- Irregular and chaotic movement.
- High turbulence.
- Common in natural streams and rivers.
- Factors: Influenced by obstacles, high velocities, and changes in channel geometry.
- Effect: Enhanced mixing between layers, leading to increased erosion and sediment transport capacity.
2. What is a longitudinal profile:
- A longitudinal profile represents a cross-sectional view of a stream or river channel from its source (headwaters) to its mouth (where it joins another body of water). It depicts changes in elevation along the length of the channel and provides insights into the slope, gradient, and overall morphology of the stream.
3. Changes in channel width, depth, flow velocity, and discharge between headwaters and mouth of a stream:
- Channel Width: Typically increases from headwaters to mouth. This occurs as additional tributaries join the main channel, contributing to increased water volume and channel width.
- Channel Depth: Generally increases downstream due to the accumulation of water from tributaries and increased discharge. Additionally, deeper channels are capable of carrying larger volumes of water.
- Flow Velocity: Initially higher in headwaters due to steeper gradients and narrower channels. However, as the stream progresses downstream, flow velocity may increase due to the larger channel dimensions and increased discharge compensating for reduced gradient.
- Discharge: Increases downstream as additional tributaries contribute water to the main channel. Discharge is calculated as the product of channel cross-sectional area and flow velocity, thus reflecting the combined effects of channel width, depth, and flow velocity.
4. Explanation for changes:
- Gradient Decrease: The reduction in channel gradient downstream leads to decreased energy available for erosion, resulting in a decrease in flow velocity. However, the increase in channel dimensions and discharge downstream compensates for this reduction, maintaining or even increasing flow velocity.
- Sediment Deposition: As flow velocity decreases downstream, the stream's capacity to transport sediment diminishes, leading to sediment deposition. This deposition contributes to the widening and deepening of the channel.
- Tributary Contributions: Additional water inputs from tributaries contribute to increased discharge and channel dimensions downstream. As a result, channel width, depth, and discharge generally increase from headwaters to mouth.
16.4 Running Water
Stream Erosion: Stream erosion refers to the process by which running water removes soil and weathered rock from the Earth's surface, primarily through the action of flowing water.
Raindrop Erosion: Raindrops dislodge sediment particles, initiating erosion, particularly on saturated ground where water cannot infiltrate, leading to sheet flow and the formation of small channels or rills.
Channel Erosion: Confined flow in a channel enhances erosion, influenced by slope, discharge, and the relative resistance of bank and bed material. Unconsolidated materials are more easily eroded than bedrock.
Types of Channel Erosion:
- Quarrying: Removal of blocks from the stream bed, aided by fracturing and weathering, mainly due to impact forces exerted by flowing water.
- Abrasion: Bed and banks of a channel are bombarded by sediment particles carried by the flow, leading to erosion and smoothing of sediment grains.
- Corrosion: Soluble rock, such as limestone, is gradually dissolved by flowing water, a chemical weathering process.
Transport of Sediment:
Definition: The process by which streams carry and transport sediment, which includes dissolved, suspended, and bed loads.
Dissolved Load: Consists of minerals dissolved in water, acquired from groundwater and precipitation, with velocity having minimal effect on transport.
Suspended Load: Mainly comprises fine sand, silt, and clay particles carried in suspension, influenced by flow velocity and settling velocity. Settling velocity determines whether particles remain suspended or settle to the streambed.
Bed Load: Sediment too large to be carried in suspension moves along the streambed through rolling, sliding, or saltation, influenced by channel characteristics and discharge.
Capacity and Competence:
Capacity: Maximum load of solid particles a stream can transport per unit time, influenced by discharge. Large rivers with high flow velocities have large capacities.
Competence: Measure of a stream's ability to transport particles based on size, determined by flow velocity. Higher velocity increases competence exponentially, affecting the transport of larger particles.
Deposition of Sediment:
Definition: Deposition refers to the process by which sediment carried by streams settles out of the flowing water, primarily due to a reduction in flow velocity.
Sorting: Sediment particles of various sizes are separated during transport, with larger particles settling first as flow velocity decreases. This process leads to the formation of depositional features composed of sorted sediment.
Alluvium: General term for sediment deposited by streams, forming various depositional features within stream channels, on the valley floor, and at the mouth of the stream.
16.4 Review
- Describe two processes by which streams cut channels in bedrock.Answer: Streams cut channels in bedrock through quarrying, involving the removal of blocks from the stream bed aided by fracturing and weathering, and abrasion, where the bed and banks are eroded by sediment particles carried by the flow.
- In what three ways does a stream transport its load? Which part of the load moves most slowly?Answer: A stream transports its load through dissolved load (minerals dissolved in water), suspended load (fine particles carried in suspension), and bed load (coarser sediment moving along the streambed). The bed load moves most slowly.
- What is settling velocity? What factors influence settling velocity? Does settling velocity affect the dissolved load?Answer: Settling velocity is the speed at which a particle falls through still water. Factors influencing settling velocity include particle size, shape, and specific gravity. Settling velocity primarily affects the transport of suspended and bed loads, not the dissolved load.
16.5 Stream Channels
1. Bedrock Channels:
- Definition: Channels cut into solid rock, typically forming in headwaters with steep slopes.
- Characteristics:
- Energetic flow transports coarse particles that actively abrade the channel.
- May feature steps (steep segments with rapids) and pools (flatter segments with accumulated alluvium).
- Channel pattern often controlled by underlying geologic structure, resulting in winding or irregular channels.
- Example: Streams flowing over steep terrain in mountainous regions.
2. Alluvial Channels:
- Definition: Channels composed mainly of unconsolidated sediment (alluvium) and undergo significant changes in shape due to erosion, transportation, and deposition of sediments.
- Characteristics:
- Shape influenced by sediment size, channel gradient, and discharge.
- Two common types: meandering channels and braided channels.
- Example: Many lowland rivers and streams with gentle gradients.
3. Meandering Channels:
- Definition: Streams characterized by sweeping bends called meanders, flowing in relatively deep, smooth channels and primarily transporting mud, sand, and fine gravel.
- Characteristics:
- Meanders evolve over time as bends migrate across the floodplain.
- Erosion focused at the outside of meanders (cut bank), while sediment deposition occurs on the inside (point bars).
- Bends migrate laterally and downstream over time, forming oxbow lakes through cutoff processes.
- Example: Lower Mississippi River.
4. Braided Channels:
- Definition: Complex network of converging and diverging channels threading among islands or gravel bars, formed where a large portion of a stream’s sediment load consists of coarse material and the stream has a highly variable discharge.
- Characteristics:
- Channels wide and shallow due to erodible bank material.
- Form in environments with high sediment supply and variable flow rates.
- Sediment deposition forms bars, causing flow to split into multiple paths around them.
- Example: Streams at the terminus of glaciers with large seasonal discharge variations.
Key Points:
- Bedrock channels cut into solid rock, often in headwaters with steep slopes, while alluvial channels are composed of unconsolidated sediment and undergo shape changes.
- Meandering channels feature sweeping bends (meanders) and lateral migration, while braided channels consist of multiple, interwoven paths separated by bars.
- Channel characteristics influenced by factors such as sediment size, channel gradient, discharge, and underlying geologic structure.
- Understanding stream channel types helps explain landscape formation and erosion processes.
16.5 Review Questions
1. Are bedrock channels more likely to be found near the head or the mouth of a stream?
Bedrock channels are more likely to be found near the head of a stream, especially in the headwaters where streams have steep slopes. As streams flow downstream and encounter gentler gradients, they are more likely to transition to alluvial channels composed of sediment rather than cutting into solid rock.
2. Describe or sketch the evolution of a meander, including how an oxbow lake forms.
- Initially, a straight channel may develop a bend due to erosion on one bank and deposition on the opposite bank.
- Over time, the bend becomes more pronounced as erosion continues on the outer bank (cut bank) and sediment deposition occurs on the inner bank (point bar).
- As the bend migrates laterally downstream, it may eventually loop around to form an oxbow-shaped meander.
- During high-flow events, the neck of the meander may be breached, leading to the formation of a cutoff, isolating the oxbow-shaped segment from the main channel and forming an oxbow lake.
3. Describe a situation that might cause a stream channel to become braided.
A stream channel might become braided in situations where there is a high sediment supply, variable flow rates, and erodible bank material. For example:
- Glacial meltwater streams at the terminus of glaciers, where large volumes of sediment are delivered seasonally.
- Streams with highly variable discharge patterns, such as those in arid or semi-arid regions experiencing flash floods.
- Environments where there are abundant sediment sources, such as in areas with active erosion or deposition processes.
Stream Erosion (Notes from Instructor)
Lecture #12: Stream Erosion
Stream - A body of running water that is confined in a channel and moves downhill under the influence of gravity. A flood occurs when the stream fills and leaves its channel.
Channel - A long narrow depression eroded by the stream into rock or sediment.
Flood plain - A broad strip of land built up by sedimentation on either side of a stream channel.
Sheetwash - A thin layer of unchanneled water flowing downhill.
Discharge - The amount of volume of water that flows past a given point in a unit time.
Gradient - Downhill slope of the stream bed. Changes in gradient can cause deposition and erosion.
Runoff - The average annual rainfall on the area of the United States is equivalent to a layer of water 76 cm thick covering this same land surface. Of this layer, 45 cm returns to the atmosphere by evaporation and transpiration and 1 cm infiltrates the ground; the remaining 30 cm forms runoff, the portion of precipitation that flows over the land surface.
Base level - The limiting level below which a stream can not erode the land. As a stream flows downslope, its potential energy decreases and finally falls to zero as it reached the sea.
Types of Channels:
Meandering Channels - a channel which forms a series of smooth bends that are similar in size and resemble in shape the switch backs of a mountain road.
Meander cutoff - a new shorter channel across the narrow neck of a meander. Nearly 600 km of the Mississippi River channel has been abandoned since 1776 by meander cutoff. However, the river has not been shortened appreciably because the loss of channel due to cutoffs has been balanced by lengthening of the channel as other meanders have enlarged.
Oxbow lake - a lake within a cutoff meander.
Braided Channels - A stream which has lost its main channel by the deposition of bars. Usually such streams are heavily loaded. The stream widens continuously as more bars are deposited.
Stream's Load - Sediment load transported by a stream can be subdivided into bed load, suspended load, and dissolved load.
Bed Load - Heavy or large sediment particles that travel near or on the stream bed. These particles are either sand or gravel and they move by saltation (a series of short leaps or bounces of the bottom).
Suspended Load - Sediment that is light enough to remain lifted indefinitely above the bottom by water turbulence.
Dissolved Load - Soluble products of chemical weathering.
Stream Deposits:
Bar - a ridge of sediment, usually sand or gravel, deposited in the middle or along the banks of a stream.
Flood plain - part of a stream valley which is inundated by floodwater.
Levee - a broad, low ridge of fine alluvium build along the side of a channel by debris -laden flood water.
Terraces - the remnant of abandoned flood plains.
Alluvial Fan - a fan-shaped body of alluvium typically built where a stream leaves a steep mountain valley.
Deltas - a sedimentary deposit that forms where a stream flows into standing water and so-named because it may develop a crudely triangular shape that resembles the Greek letter delta.
Stream Patterns: - A tributary is a smaller stream joining the main stream at an acute angle. The nature by which tributaries join main streams determines the drainage pattern.
Dendritic - irregular branching of channels in many directions.
Trellised - Rectangular arrangement of channels in which principal tributary streams are parallel and very long.
Parallel - Parallel or subparallel channels that have formed on sloping surfaces underlain by homogeneous rocks.
Radial - channels radiate out, like the spokes of a wheel from a topographically high area.
Rectangular - channel system marked by right-angel bends.
16.6 Stream Valleys
Stream Valleys:
- Definition: Stream valleys consist of the channel and surrounding terrain that directs water into a stream, including the valley floor and walls.
- Characteristics: Vary from narrow, steep-sided valleys to wide, flat valleys; affected by stream erosion and weathering.
Valley Deepening:
- Description: Occurs when a stream's gradient is steep, resulting in downcutting and the formation of V-shaped valleys.
- Features: Rapids and waterfalls are prominent in V-shaped valleys, formed by variations in bedrock erodibility.
Valley Widening:
- Description: Occurs as streams approach graded conditions, leading to lateral erosion and meandering patterns.
- Features: Floodplains develop as streams widen their valleys, covering the valley floor with alluvium.
Incised Meanders and Stream Terraces:
- Incised Meanders: Meanders in steep, narrow bedrock valleys formed due to changes in base level, causing downcutting.
- Stream Terraces: Formed when a river adjusts to a relative drop in base level, leaving remnants of former floodplains as flat surfaces above newly forming floodplains.
Key Concepts:
- Base Level: Lowest elevation to which a stream can erode its channel; includes ultimate base level (sea level) and local base levels.
- Graded Streams: Streams with the necessary slope and characteristics to maintain sediment transport without erosion or deposition.
- Floodplain: Broad, flat valley floor covered with alluvium, inundated during flood stage.
- Erosional vs. Depositional Floodplains: Erosional floodplains form through lateral erosion, while depositional floodplains result from major fluctuations in conditions.
- Slot Canyons: Narrow valleys with vertical walls, often found in arid regions with resistant rock.
The base level upstream from the reservoir is raised, which reduces the stream’s flow velocity and leads to deposition and a reduced gradient.
A resistant layer of rock can act as a temporary base level. The stream concentrates its erosive energy on the resistant rock at the knickpoint. Eventually the river eliminates the knickpoint and reestablishes a smooth profile.
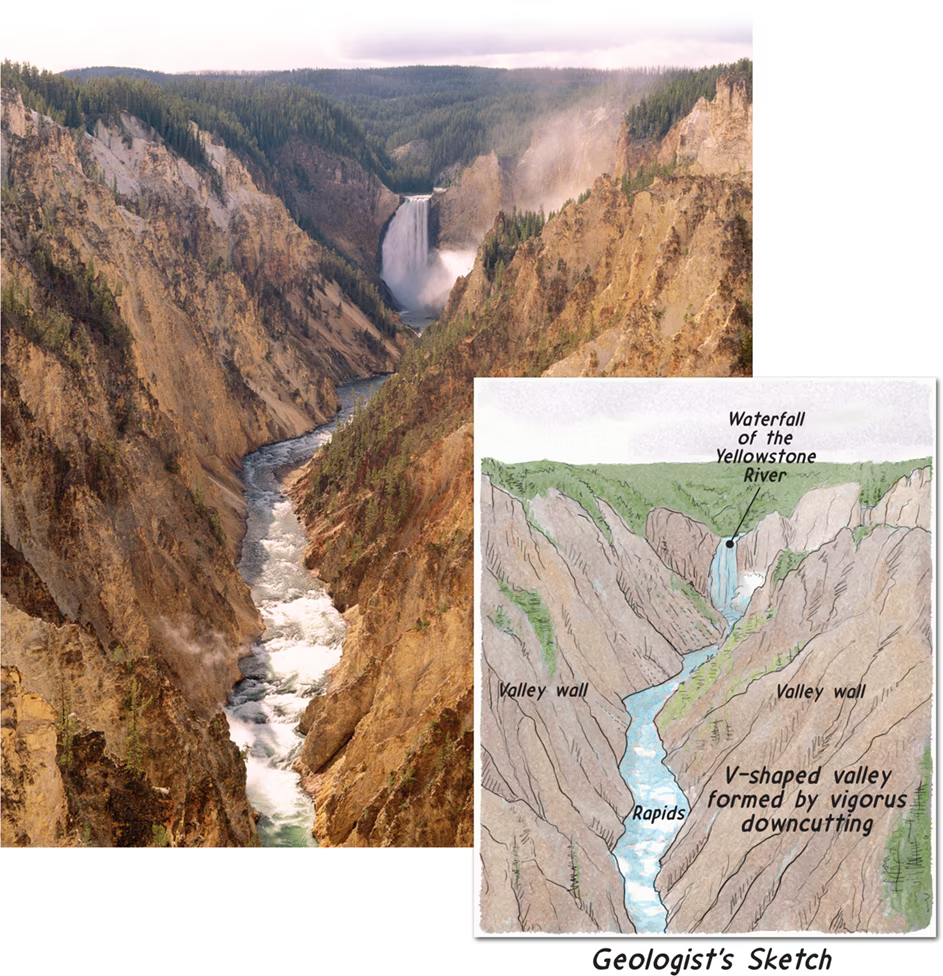
The V-shaped valley, rapids, and waterfalls indicate that the river is vigorously downcutting.
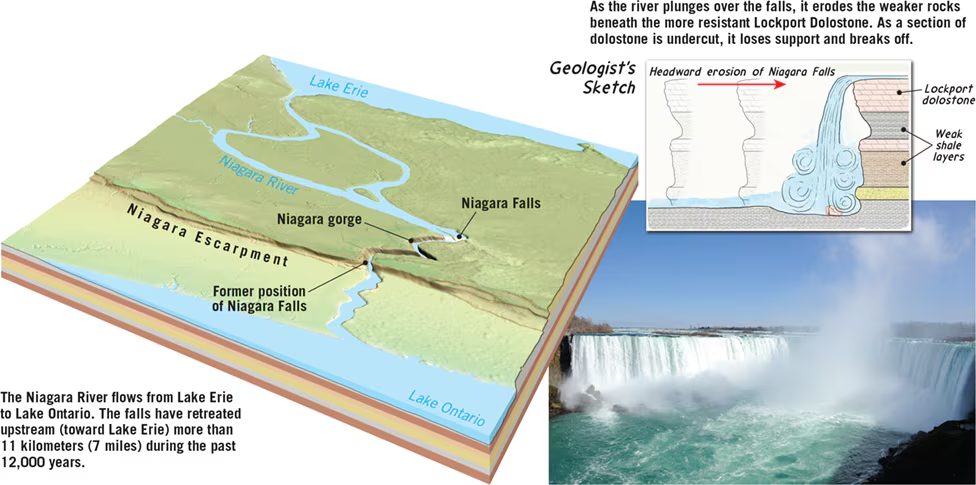
Continuous side-to-side erosion by shifting meanders gradually produces a broad, flat valley floor. Alluvium deposited during floods covers the valley floor.
16.6 Review Questions
1. Define base level and distinguish between ultimate base level and local, or temporary, base level.
- Question: What is base level, and how does it vary?
- Answer: Base level is the lowest point to which a stream can erode its channel. Ultimate base level refers to sea level, while local base level includes lakes, resistant rock layers, or other rivers acting as temporary limits to erosion.
2. Explain why V-shaped valleys often contain rapids and/or waterfalls.
- Question: What features are common in V-shaped valleys, and why?
- Answer: V-shaped valleys result from steep downcutting by streams. Rapids and waterfalls occur where streams encounter resistant rock layers, creating variations in gradient and flow velocity.
3. List two situations that would trigger the formation of incised meanders.
- Question: What conditions lead to the formation of incised meanders?
- Answer: Incised meanders form when there is a relative drop in base level due to either a drop in downstream base level or regional land uplift, causing streams to downcut and meander in narrow bedrock valleys.
16.7 Depositional Landforms
1. Deltas:
- Definition: Landforms formed at the mouth of rivers where sediment-laden streams enter still waters such as lakes, inland seas, or oceans.
- Formation Process:
- Sediments are deposited as the stream's forward motion slows upon entering the still water, forming foreset, topset, and bottomset beds.
- The delta grows outward as sediment accumulates, causing the stream's gradient to decrease and leading to the formation of distributaries.
- Deltas vary in shape and structure based on shoreline configurations, wave activity, and sediment supply.
- Example: The Mississippi River Delta, characterized by multiple subdeltas and a bird-foot delta shape.
2. Natural Levees:
- Definition: Elevated ridges parallel to river channels, formed by successive floods depositing coarse sediment adjacent to the channel.
- Formation Process:
- During floods, coarse sediments settle near the channel due to decreased flow velocity, forming elevated ridges.
- Over time, successive floods build up these ridges, creating natural levees that rise above the adjacent floodplain.
- Importance: Provide flood protection and influence drainage patterns, often leading to the formation of back swamps and yazoo tributaries.
3. Alluvial Fans:
- Definition: Fan-shaped deposits of sediment formed at the base of steep mountain slopes when high-gradient streams suddenly emerge onto flat plains.
- Formation Process:
- Streams deposit sediment rapidly due to the sudden decrease in velocity upon exiting narrow valleys, forming a cone- or fan-shaped accumulation.
- Coarser material settles near the apex of the fan, while finer material is carried toward the base.
- Example: Death Valley in California, known for its large alluvial fans formed by intermittent water flow during rainy periods.
Key Concepts:
- Deltas form at the mouths of rivers and are characterized by sediment deposition into still waters, creating distributaries and varying in shape based on sediment supply and wave activity.
- Natural levees are raised ridges parallel to river channels, formed by successive floods depositing coarse sediment adjacent to the channel.
- Alluvial fans develop at the bases of steep mountain slopes, where high-gradient streams deposit sediment rapidly upon exiting narrow valleys, forming cone- or fan-shaped accumulations.
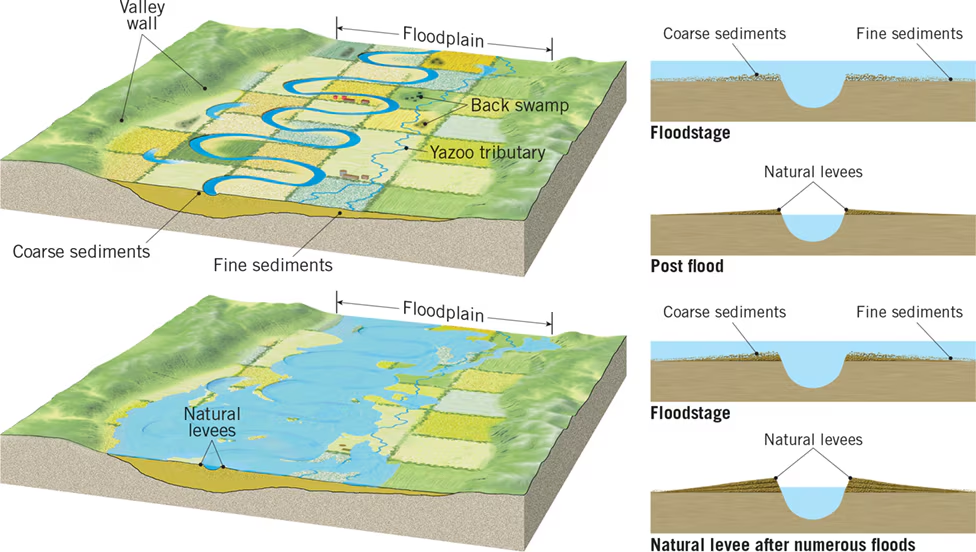
These gently sloping structures that parallel a river channel are created by repeated floods. Because the ground next to the channel is higher than the adjacent floodplain, back swamps and yazoo tributaries may develop.
Fans are deposited at the mouth of a valley that emerges from a mountainous or
upland area onto a relatively flat lowland. Usually coarse material is dropped near the apex of the fan, while finer materials are carried toward the base of the deposit. California’s Death Valley has many large fans. As adjacent fans grow larger, they may coalesce to form a steep apron of sediment called a bajada.
16.7 Review Questions
1. **Sketch a cross section of a simple delta and distinguish among the three types of beds that compose it.**
- **Question:** How would you sketch a cross section of a simple delta, and what are the three types of beds that make up its structure?
- **Answer:** A simple delta cross section consists of foreset beds (coarse particles from the bed load deposited downcurrent from the delta front), topset beds (deposited during flood stage), and bottomset beds (fine sediments settled away from the mouth in horizontal layers).
2. **Briefly describe the formation of a natural levee. How is this feature related to back swamps and yazoo tributaries?**
- **Question:** How do natural levees form, and what is their relationship to back swamps and yazoo tributaries?
- **Answer:** Natural levees form when successive floods deposit coarse sediment adjacent to a river channel during overflow, creating elevated ridges. Back swamps develop behind levees due to poor drainage, and yazoo tributaries flow through back swamps before rejoining the main river channel.
3. **Describe the formation of an alluvial fan.**
- **Question:** How does an alluvial fan form?
- **Answer:** An alluvial fan forms at the base of steep mountain slopes when high-gradient streams exit narrow valleys onto flat plains. The sudden decrease in velocity causes rapid sediment deposition, forming cone- or fan-shaped accumulations.
Chapter 17
17.1 The Importance of Groundwater
1. Groundwater as a Freshwater Source:
- Definition: Water stored beneath the Earth's surface in pore spaces and fractures.
- Importance:
- Represents the largest reservoir of readily available freshwater.
- Comprises about 30% of Earth's freshwater, excluding ice.
- Essential for sustaining human populations, agriculture, and ecosystems.
2. Geologic Role of Groundwater:
- Erosional Agent:
- Dissolves soluble rock, such as limestone, forming sinkholes and caverns.
- Equalizes Streamflow:
- Provides storage and sustains streamflow during dry periods by slowly releasing water to stream channels.
3. Groundwater as a Basic Resource:
- Usage:
- Supplies approximately 25% of freshwater in the United States.
- Widely used for irrigation, public/domestic, aquaculture, and industrial purposes.
- Advantages:
- Availability in areas lacking reliable surface water sources.
- Stored in subsurface pore spaces and fractures, providing a continuous supply.
4. Primary Uses of Groundwater:
- Irrigation: Largest use, supporting agriculture in arid regions.
- Public and Domestic: Indoor and outdoor household purposes, including drinking, cooking, bathing, and watering lawns.
- Aquaculture: Water for fish and shellfish farms.
- Industrial: Significant in mining, petroleum refining, and various manufacturing processes.
5. Trends in Water Use:
- Changing Demands: Growing population, increased industry, and agricultural needs.
- Conservation Efforts: Water use in the United States has decreased despite population growth, due to conservation and efficiency measures.
- Challenges: Some regions experiencing declining groundwater levels due to excessive extraction.
6. Environmental and Economic Importance:
- Ecosystem Support: Vital for maintaining habitats and biodiversity.
- Economic Impact: Essential for various industries and agricultural productivity.
- Conservation: Importance of sustainable management practices to ensure long-term availability.
7. Conclusion:
- Groundwater plays a critical role in sustaining life, ecosystems, and economies worldwide.
- Balancing human needs with environmental preservation is essential for the future of groundwater resources.
17.1 Review
- What percentage of Earth’s total freshwater supply is groundwater?
- Groundwater accounts for approximately 30% of Earth's total freshwater supply.
- What share of Earth’s liquid freshwater is groundwater?
- Groundwater represents approximately 96% of Earth's liquid freshwater.
- List two geologic roles that groundwater plays.
- Groundwater serves as an erosional agent by slowly dissolving soluble rock like limestone, forming features such as sinkholes and caverns.
- It helps equalize streamflow by providing sustained water flow during dry periods, thus contributing to the erosion and shaping of stream valleys.
- What share of U.S. freshwater is provided by groundwater?
- Groundwater provides about 25% of the total freshwater used in the United States.
- What is most groundwater used for?
- The majority of groundwater is used for irrigation purposes, particularly in agriculture.
17.2
1. Distribution of Water Beneath Earth's Surface:
- Rainfall paths: Some runoff, some evaporation/transpiration, some infiltration.
- Factors affecting infiltration: Slope steepness, surface material type, rainfall intensity, vegetation density and type.
- Zones:
- Soil Moisture Zone: Near-surface area where water is held by soil particles and used by plants.
- Zone of Saturation: Area where all spaces in sediment and rock are filled with water (groundwater).
- Capillary Fringe: Transitional zone above the water table where groundwater is held by surface tension.
- Unsaturated Zone: Area above the water table where pore spaces contain both air and water.
2. Zone of Saturation and Water Table:
- Zone of Saturation: Contains groundwater, where all pores in sediment and rock are filled with water.
- Water Table: Upper boundary of the zone of saturation; fluctuates due to factors like precipitation and pumping.
- Capillary Fringe: Layer above the water table where groundwater is drawn upward by capillary action.
- Unsaturated Zone: Area above the water table where soil pores contain air and some water.
3. Variations in the Water Table:
- Highly variable due to factors such as precipitation, geology, and human activities.
- Seasonal and yearly fluctuations depending on precipitation patterns.
- Monitored and mapped through well water levels, revealing irregular surface topography replication.
4. Factors Contributing to Water Table Variability:
- Groundwater Movement: Varies in rate, influencing water accumulation beneath high areas.
- Precipitation and Permeability: Varied precipitation and surface permeability lead to uneven water table levels.
- Human Activities: Pumping groundwater for irrigation or drinking can also alter the water table.
5. Interactions Between Groundwater and Streams:
- Gaining Streams: Receive water from groundwater inflow through streambeds.
- Losing Streams: Lose water to groundwater outflow through streambeds.
- Combined Interaction: Some sections of streams gain water, while others lose it.
- Groundwater Contribution to Streams: Groundwater is a major source of water for streams, influencing their flow and stability.
This detailed study guide covers the distribution of water beneath Earth's surface, characteristics of the zone of saturation and water table, variations in the water table, factors contributing to its variability, and interactions between groundwater and streams.
17.2 Review Questions
- When rain falls on land, what factors influence the amount of water that soaks in?
- Factors such as slope steepness, surface material type, rainfall intensity, and vegetation density and type influence the amount of water that infiltrates into the ground.
- Define groundwater and relate it to the water table.
- Groundwater is water that fills the spaces in sediment and rock beneath Earth's surface. It is closely related to the water table, which is the upper boundary of the zone of saturation where groundwater is found.
- A kitchen table is flat. Is this usually the case for a water table? Why?
- No, the water table is not usually flat like a kitchen table. It follows the topography of the land surface, meaning it is typically higher beneath hills and lower in valleys.
- Contrast a gaining stream and a losing stream.
- A gaining stream receives water from groundwater inflow through its streambed, while a losing stream loses water to the groundwater system through its streambed.
17.3 Storage and Movement of Groundwater
1. Factors Influencing Groundwater Storage and Movement:
- Porosity:
- Definition: Porosity refers to the percentage of the total volume of rock or sediment that consists of pore spaces.
- Influential Factors: Grain size, sorting, packing, and cementation affect porosity.
- Permeability:
- Definition: Permeability is a measure of a material's ability to transmit fluids and is crucial for groundwater movement.
- Influential Factors: Porosity alone does not indicate permeability; connectedness and size of pores are essential.
- Aquitards and Aquifers:
- Aquitards: Impermeable layers hinder groundwater movement.
- Aquifers: Permeable rock strata or sediments that transmit groundwater freely are termed aquifers.
2. Nature of Groundwater Movement:
- Slow Movement: Contrary to surface water, groundwater movement is slow, primarily occurring from pore to pore.
- Flow Paths: Groundwater moves along flow paths from recharge areas to discharge areas, such as springs, lakes, streams, and coastal areas.
3. Measuring Groundwater Movement:
- Henri Darcy's Experiments: In the mid-19th century, Darcy's experiments established principles of groundwater movement.
- Hydraulic Gradient: The slope of the water table determines groundwater velocity, expressed as the hydraulic gradient.
- Hydraulic Conductivity: Defined by Darcy, it represents the permeability of aquifers and influences flow rate.
- Darcy's Law: An equation used to determine discharge through an aquifer based on hydraulic gradient, hydraulic conductivity, and cross-sectional area.
4. Different Scales of Movement:
- Geographic Extent: Groundwater flow systems vary in size from local to regional scales.
- Flow Paths: Range from meters to kilometers, with complex interactions between shallow and deep systems.
- Cross-Sectional Diagram: Illustrates the complex arrangement of aquifers and aquitards, showing local and regional flow systems.
Key Points:
- Porosity and permeability are critical factors influencing groundwater storage and movement.
- Groundwater moves slowly from recharge to discharge areas along flow paths.
- Darcy's experiments laid the foundation for understanding groundwater movement, including hydraulic gradient and conductivity.
- Groundwater movement occurs at various scales, from local to regional, with complex interactions between different systems.
- Groundwater flows more rapidly through sediments having greater permeability than through materials having lower permeability. He defined a coefficient known as hydraulic conductivity that takes into account the permeability of the aquifer and the viscosity of the fluid. To determine discharge (Q)—that is, the actual volume of water that flows through an aquifer in a specified time—the following equation is used:where is the hydraulic gradient, K is the coefficient that represents hydraulic conductivity, and A is the cross-sectional area of the aquifer. This expression has come to be called Darcy’s law. Using this equation, if you know an aquifer’s hydraulic gradient, conductivity, and cross-sectional area, you can calculate its discharge.
Q= K A (H1-2)
d
The hydraulic gradient is determined by measuring the difference in elevation between two points on the water table divided by the distance between them, d. Wells are used to determine the height of the water table.
17.3 Review Questions
- Distinguish between porosity and permeability.
Porosity refers to the percentage of the total volume of rock or sediment that consists of pore spaces, while permeability is a measure of a material's ability to transmit fluids. Porosity indicates the storage capacity of groundwater, whereas permeability determines the ease with which water can flow through a material.
- What is the difference between an aquifer and an aquitard?
An aquifer is a permeable rock stratum or sediment that transmits groundwater freely, allowing significant water movement. Conversely, an aquitard is an impermeable layer that hinders or prevents water movement, acting as a barrier to groundwater flow.
- What factors cause water to follow the paths shown in Figure 17.10?
Water follows paths depicted in Figure 17.10 due to gravitational forces and pressure differentials. Groundwater moves from areas of higher elevation (recharge areas) to lower elevation (discharge areas), following the hydraulic gradient. Additionally, permeability variations in subsurface materials influence the direction and speed of groundwater movement.
- Relate groundwater movement to hydraulic gradient and hydraulic conductivity.
Groundwater movement is related to both hydraulic gradient and hydraulic conductivity. The hydraulic gradient, determined by the slope of the water table, governs the direction and velocity of groundwater flow. Hydraulic conductivity, a measure of a material's ability to transmit fluids, influences the rate of groundwater movement through porous media. Together, these factors determine how groundwater flows within an aquifer system.
17.4 Study Guide: Wells and Artesian Systems
- Wells:
- Definition: Wells are holes bored into the zone of saturation to extract groundwater, serving as small reservoirs for water migration and extraction.
- Significance: Groundwater from wells is a crucial source of drinking water, providing for approximately half the U.S. population and 96 percent of rural areas.
- Water Table and Wells:
- Fluctuations: The water table fluctuates seasonally, necessitating wells to penetrate below the water table for continuous water supply.
- Drawdown and Cone of Depression: Drawdown occurs when water is withdrawn from a well, creating a depression in the water table known as a cone of depression. This effect increases the hydraulic gradient near the well.
- Challenges of Well Drilling:
- Heterogeneous Subsurface Materials: Variability in subsurface materials leads to varying success rates in drilling wells. Perched water tables and geological structures impact the availability of groundwater.
- Artesian Systems:
- Definition: Artesian systems involve groundwater under pressure rising above the level of the aquifer, often leading to flowing artesian wells or springs.
- Conditions: Artesian systems require an inclined aquifer confined by impermeable layers (aquitards) above and below, creating confined aquifers.
- Flowing vs. Non-flowing Wells: Depending on the pressure surface's position relative to the ground level, wells in artesian systems can be flowing or non-flowing.
- Transmitting Water: Artesian systems act as conduits, transporting water over long distances from recharge areas to discharge points, influencing landscapes and ecosystems.
- Examples of Artesian Systems:
- Natural Springs: Artesian springs can emerge along natural fractures, contributing to oases in desert regions.
- Human Impact: Human activities, such as extensive well drilling, can deplete artesian systems, leading to decreased water flow and lower water tables.
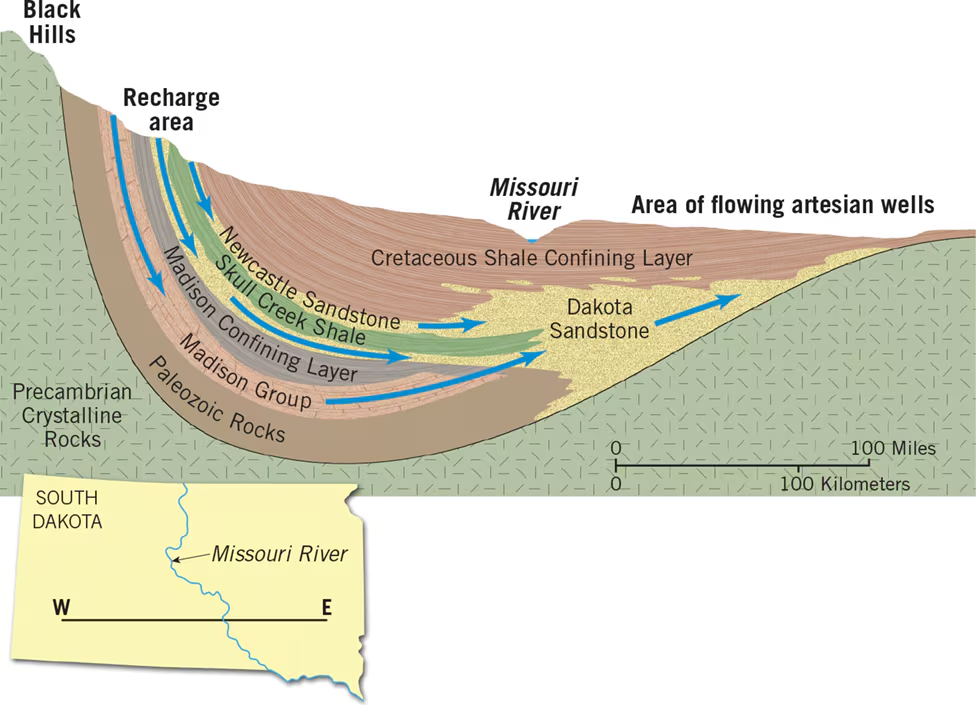
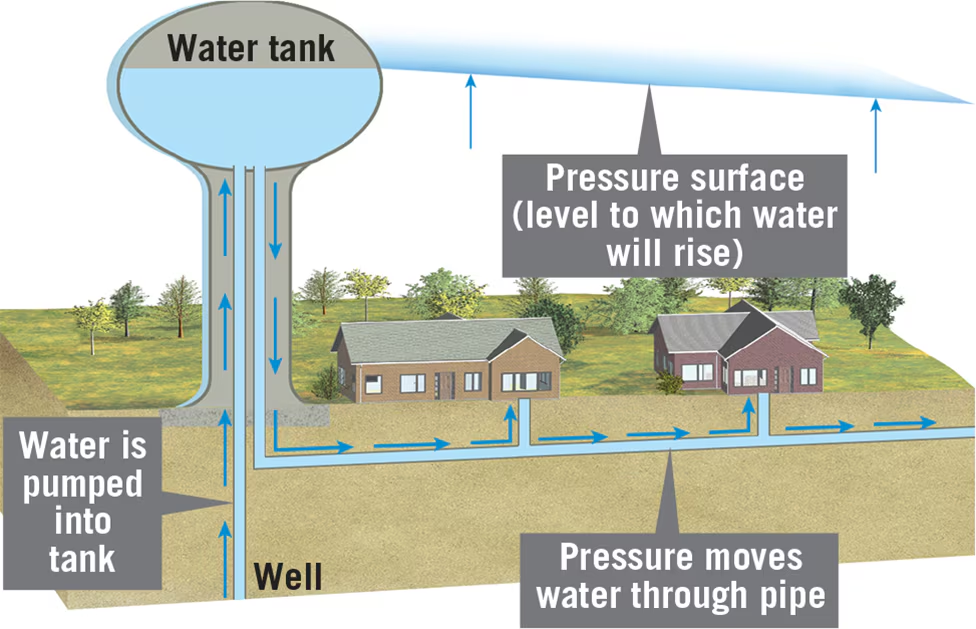
17.4 Review Questions
1. Define drawdown and relate this term to the term cone of depression.
- Drawdown: Drawdown refers to the lowering of the water table around a well when water is withdrawn, creating a depression in the water table.
- Relation to Cone of Depression: Drawdown contributes to the formation of a cone of depression, which is a roughly conical-shaped depression in the water table around a heavily pumped well. The drawdown decreases with distance from the well, resulting in a depression that resembles a cone.
2. Sketch a simple cross-section of an artesian system with a flowing well. Label aquitards, aquifers, and the pressure surface.
[Sketch]
- Aquitards: Impermeable layers above and below the aquifer.
- Aquifers: Permeable layers that transmit groundwater.
- Pressure Surface: Represents the level to which water would rise if not confined.
3. Why do some artesian wells not flow at Earth’s surface?
- Frictional Losses: Friction between the flowing water and the surrounding rock reduces the pressure, causing the water level to drop before reaching the surface.
- Hydraulic Gradient: Greater distance from the recharge area increases friction, resulting in less pressure and reduced flow at the surface.
- Depleted Reservoirs: Overuse of the aquifer can deplete the reservoir, lowering the water table and reducing pressure, leading to non-flowing artesian wells.
17.6 Environmental Problems Associated with Groundwater
- Overexploitation and Nonrenewable Resource Treatment:
- Groundwater is increasingly exploited, leading to overuse in some areas.
- In regions where withdrawal exceeds recharge, groundwater is essentially mined, causing long-term imbalances in the water table.
- Land Subsidence Due to Groundwater Withdrawal:
- Excessive pumping causes the ground to sink as the weight of overlying sediments compresses due to decreased water pressure.
- Examples include the San Joaquin Valley in California, southern Arizona, Las Vegas, New Orleans, and Houston.
- Impact of Prolonged Drought:
- Drought increases groundwater usage, leading to land subsidence.
- California's severe drought in 2016 resulted in significant land subsidence due to increased groundwater pumping.
- Saltwater Intrusion:
- Coastal areas face the threat of saltwater encroachment into freshwater aquifers.
- Over-pumping lowers the water table, allowing saltwater to intrude into wells, contaminating freshwater supplies.
- Groundwater Contamination:
- Pollution from sewage, septic tanks, broken sewer systems, and farm wastes threatens groundwater quality.
- Hazardous chemicals, fertilizers, pesticides, and industrial materials also contribute to contamination.
- Slow groundwater movement can delay detection of pollution until after drinking water is affected.
- Prevention is the most effective solution to groundwater contamination.
Important Details:
- High Plains Aquifer: Example of severe groundwater depletion due to intense irrigation and limited recharge.
- Land Subsidence Examples: San Joaquin Valley in California, southern Arizona, Las Vegas, New Orleans, and Houston.
- Saltwater Intrusion: Occurs in coastal areas where over-pumping allows saltwater to contaminate freshwater aquifers.
- Impact of Prolonged Drought: California's 2016 drought led to increased groundwater pumping and land subsidence.
- Groundwater Contamination Sources: Sewage, septic tanks, broken sewer systems, farm wastes, highway salt, fertilizers, pesticides, industrial chemicals, and landfill leachate.
- Solutions: Prevention is crucial. Other solutions include abandoning contaminated water supplies, natural recharge, and pumping and treating polluted water.
17.6 Review Questions
- Describe the problem associated with pumping groundwater for irrigation in the southern High Plains.
- The problem in the southern High Plains arises from intense irrigation, primarily for agriculture, leading to excessive groundwater withdrawal. The area receives limited precipitation, resulting in minimal recharge to replenish the aquifer. As a consequence, groundwater levels decline rapidly due to overuse, risking depletion of the aquifer and posing a threat to sustainable water supply.
- Explain why the ground may subside after groundwater is pumped to the surface.
- Ground subsidence occurs when groundwater is pumped from wells at a faster rate than natural recharge processes can replenish it. As water is withdrawn, the water pressure decreases, causing the overlying sediment to compact. This compaction results in a loss of support, leading to the sinking or subsidence of the ground surface.
- Which aquifer would be most effective in purifying polluted groundwater: coarse gravel, sand, or cavernous limestone?
- The aquifer composed of cavernous limestone would be the most effective in purifying polluted groundwater. Cavernous limestone contains numerous interconnected openings and conduits, allowing groundwater to flow slowly through the aquifer. This slow flow rate provides ample time for natural processes such as mechanical filtration, chemical oxidation, and assimilation by other organisms to purify the contaminated water.
- Describe a significant problem that may arise when groundwater is heavily pumped at a coastal site.
- A significant problem that may arise when groundwater is heavily pumped at a coastal site is saltwater intrusion. Over-pumping of groundwater lowers the water table, creating a hydraulic gradient that draws saltwater from the ocean into freshwater aquifers. As a result, the intrusion of saltwater contaminates the freshwater supply, rendering it unsuitable for consumption or agricultural use. This phenomenon poses a severe threat to coastal communities dependent on groundwater for their water needs.
17.7 Geologic Work of Ground Water
Definitions:
- Caverns: Large underground chambers formed by the dissolution of soluble rocks such as limestone by groundwater.
- Karst Topography: A landscape characterized by sinkholes, caves, and underground drainage systems, formed due to the dissolution of soluble rocks like limestone by groundwater.
Important Details:
- Formation of Caverns and Development of Karst Topography:
- Groundwater dissolves soluble rocks, particularly limestone, forming caverns and contributing to the development of karst topography.
- Caverns are created by the dissolution of limestone by acidic groundwater, mainly in the zone of saturation.
- Cavern development occurs along lines of weakness in the rock, gradually enlarging cavities into interconnected passages.
- Dripstone formations, including stalactites, stalagmites, and columns, are depositional features formed by the precipitation of calcium carbonate from dripping water in caves.
- Karst Topography:
- Karst topography is characterized by irregular terrain with sinkholes, caves, and underground drainage systems.
- It occurs in areas underlain by soluble rocks like limestone, with notable examples in Kentucky, Tennessee, and Florida.
- Sinkholes are depressions formed either gradually by the dissolution of limestone or abruptly by the collapse of cavern roofs.
- Karst regions typically lack surface drainage, with runoff funneled underground through sinkholes.
- Tower Karst:
- Tower karst is a type of karst topography found in wet tropical and subtropical regions, characterized by steep-sided hills or towers rising abruptly from the ground.
- It forms in areas with thick beds of highly jointed limestone, where groundwater dissolves large volumes of rock, leaving behind residual towers.
- Examples of tower karst regions include southern China, Puerto Rico, and western Cuba.
Key Takeaways:
- Groundwater plays a significant role in shaping the Earth's surface through the dissolution of soluble rocks, leading to the formation of caverns and karst topography.
- Caverns exhibit unique dripstone formations created by the deposition of calcium carbonate from dripping water.
- Karst topography is characterized by sinkholes, caves, and underground drainage systems, with variations such as tower karst found in tropical regions.
17.7 Review Questions
How does groundwater create caverns?
- Groundwater creates caverns through a process of dissolution. When groundwater, often containing carbonic acid, comes into contact with soluble rocks like limestone, it reacts with the rock, dissolving it over time. This erosion process enlarges small cracks and fissures in the rock, gradually forming larger cavities underground.
What causes cavern formation to stop at one level (depth) but continue or begin at a lower level?
- Cavern formation often stops at one level due to changes in the water table. As streams erode deeper valleys, the water table drops, causing the groundwater level to decrease. Caverns that were forming at or just below the previous water table level may be abandoned as the water drains away. However, cavern formation can continue or begin at a lower level if the water table drops further, exposing new areas of soluble rock to the erosive action of groundwater.
How do stalactites and stalagmites form?
- Stalactites and stalagmites form in caves through the deposition of minerals carried by dripping water. Water containing dissolved calcium carbonate drips from the ceiling of a cave, leaving behind small amounts of calcite as it evaporates. Over time, these deposits accumulate, forming icicle-like structures hanging from the ceiling (stalactites) or cone-shaped mounds rising from the floor (stalagmites). Stalactites form from the ceiling downward, while stalagmites form from the floor upward.
Describe two ways in which sinkholes form.
Sinkholes can form gradually or abruptly. Gradual sinkhole formation occurs over time as rainwater, charged with carbon dioxide, seeps through the soil and dissolves the underlying limestone or other soluble rock. The bedrock surface gradually subsides, and the soil above is carried away by groundwater flow, creating shallow depressions.
- Abrupt sinkhole formation occurs when the roof of an underground cavern collapses under its own weight. This sudden collapse creates steep-sided and deep sinkholes. Such collapses can pose serious hazards, especially in populated areas.
Chapter 13
13.1 p. 365 An Emerging Picture of the Ocean Floor
1. Bathymetry:
- Definition: Bathymetry refers to the measurement of ocean depths and the mapping of the ocean floor's topography.
- Historical Perspective: Early techniques involved laboriously lowering a weighted line overboard and recording depths. The HMS Challenger's historic voyage (1872-1876) marked the first comprehensive study of the global ocean, where water depths were measured manually.
- Modern Techniques:
- Echo Sounders: Developed in the early 20th century, echo sounders transmit sound pulses (pings) into the water, measuring the time taken for the echo to return. This data is used to calculate water depth.
- Sidescan Sonar: Developed post-World War II, sidescan sonar instruments send out sound waves perpendicular to a ship's path, providing image-like representations of the seafloor.
- Multibeam Sonar: High-resolution multibeam sonar instruments, developed in the 1990s, use multiple sound beams to map wide sections of the ocean floor with detailed depth information.
2. Mapping the Ocean Floor from Space:
- Satellite Altimetry: Satellites equipped with radar altimeters measure variations in sea-surface elevation caused by gravitational forces exerted by seafloor features. This data, combined with traditional sonar measurements, aids in creating large-scale maps of the ocean floor.
3. Provinces of the Ocean Floor:
- Continental Margins: The submerged outer edge of a continent, consisting of the continental shelf, slope, and rise.
- Deep-Ocean Basins: Large areas of the seafloor that lie beyond the continental margins, characterized by abyssal plains, deep-sea trenches, and seamounts.
- Oceanic (Mid-Ocean) Ridges: Underwater mountain ranges formed by tectonic activity where new oceanic crust is created.
Key Points:
- The HMS Challenger's voyage marked the beginning of systematic bathymetric surveys.
- Modern techniques like multibeam sonar allow for high-resolution mapping of the ocean floor.
- Satellite altimetry complements traditional sonar measurements, aiding in understanding large-scale features.
- The ocean floor is divided into continental margins, deep-ocean basins, and oceanic ridges, each with distinct geological characteristics.
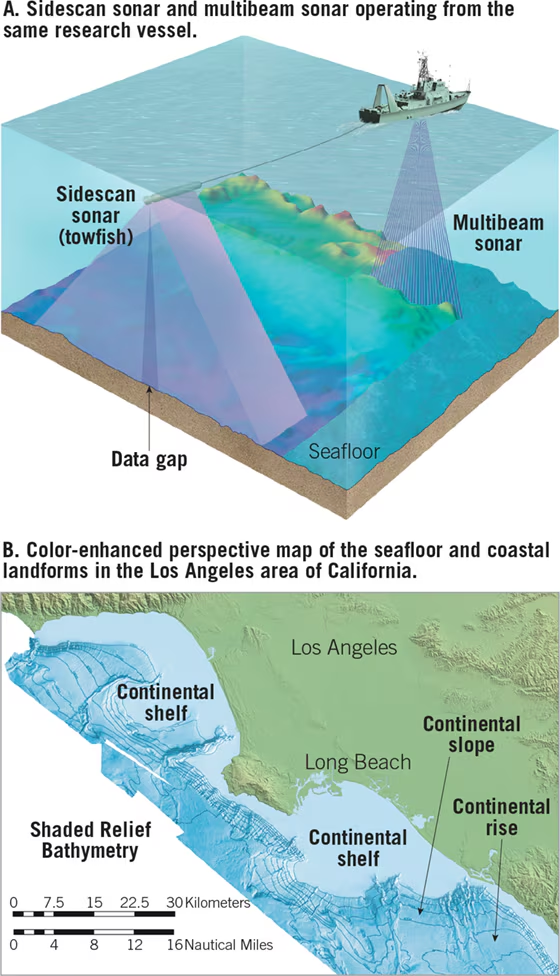
13.1 Review
- Define bathymetry: Bathymetry refers to the measurement of ocean depths and the mapping of the ocean floor's topography.
- Describe how satellites orbiting Earth can determine features on the seafloor without being able to directly observe them beneath several kilometers of seawater:
Satellites equipped with radar altimeters can measure variations in sea-surface elevation caused by gravitational forces exerted by seafloor features. By bouncing microwaves off the sea surface and compensating for factors like waves, tides, currents, and atmospheric effects, satellites can detect subtle differences in sea surface height. These variations are indicative of the underlying topography of the ocean floor. By combining this data with traditional sonar measurements, which provide direct information about the ocean floor's depth, satellites contribute to the creation of large-scale maps of the ocean floor, even though they cannot directly observe features beneath several kilometers of seawater.
- List the three major provinces of the ocean floor:
The three major provinces of the ocean floor are:
- Continental Margins
- Deep-Ocean Basins
- Oceanic (Mid-Ocean) Ridges
13.2 Continental Margins
1. Continental Margins:
- Definition: Continental margins are the outer edges of the continents where continental crust transitions to oceanic crust.
- Types of Continental Margins: Two types of continental margins exist: passive and active.
2. Passive Continental Margins:
- Definition: Passive continental margins are geologically inactive regions located far from plate boundaries.
- Major Features:
- Continental Shelf: Gently sloping, submerged surface extending from the shoreline toward the ocean basin, consisting mainly of continental crust capped with sedimentary rocks and sediments.
- Continental Slope: Relatively steep zone marking the boundary between continental crust and oceanic crust.
- Continental Rise: Gradual incline seaward from the continental slope, consisting of a thick accumulation of sediment moved down from the continental slope.
3. Active Continental Margins:
- Definition: Active continental margins are located along convergent plate boundaries where oceanic lithosphere is being subducted beneath the leading edge of a continent.
- Major Features:
- Deep-Ocean Trenches: Deep, narrow furrows formed at convergent plate boundaries where subduction occurs.
- Accretionary Wedge: Accumulation of deformed sediment and scraps of oceanic crust plastered against the edge of the overriding plate.
- Subduction Erosion: Process where sediment and rock are scraped off the bottom of the overriding plate and transported into the mantle by the subducting plate.
Key Points:
- Passive Continental Margins: Geologically inactive regions, characterized by wide continental shelves and accumulation of sediments.
- Active Continental Margins: Located at convergent plate boundaries, associated with tectonic activity such as subduction, deep-ocean trenches, and accretionary wedges.
- Distinct Features: The major features of continental margins include continental shelves, slopes, rises, deep-ocean trenches, accretionary wedges, and subduction erosion.
Distribution of subduction zones: Ring of Fire
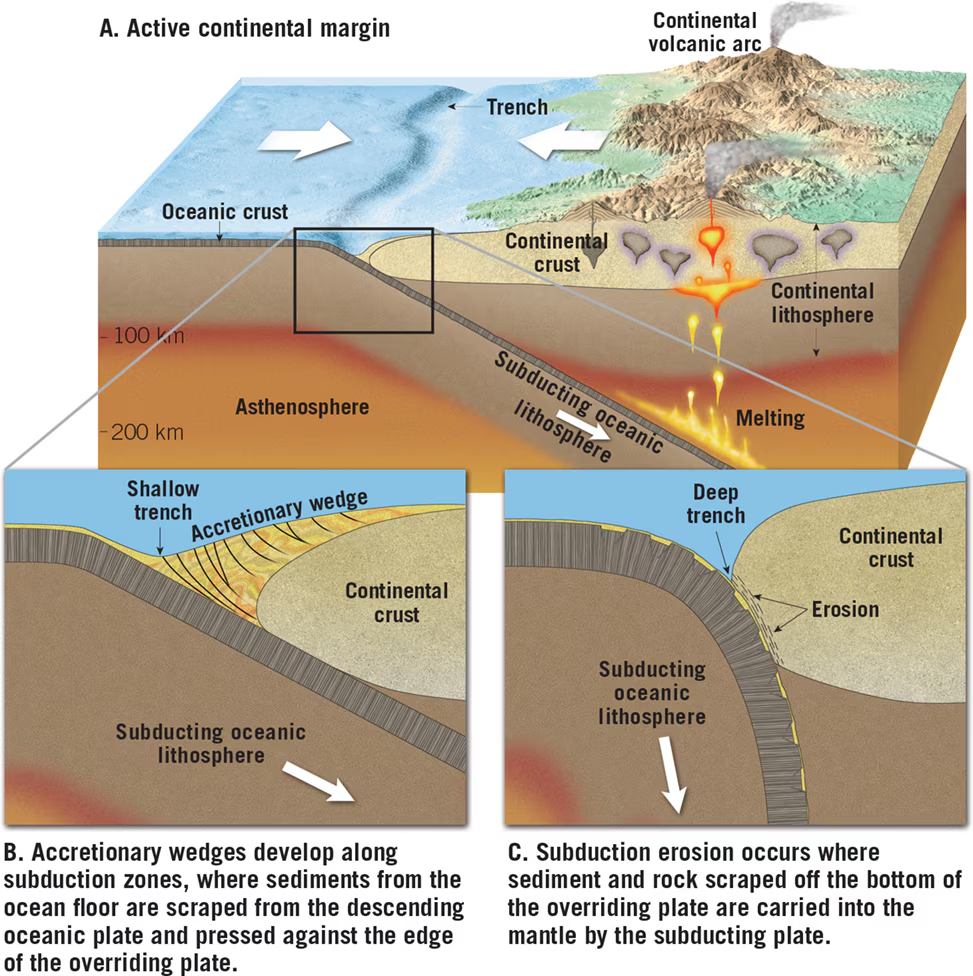
13.2 Review Questions
- List the three major features of a passive continental margin:
- Continental Shelf
- Continental Slope
- Continental Rise
- Describe the differences between active and passive continental margins. Where is each type found?
- Passive Continental Margins:
- Definition: Geologically inactive regions located far from plate boundaries.
- Major Features: Wide continental shelves, gentle continental slopes, and gradual continental rises.
- Location: Found along coastlines away from plate boundaries, such as the Atlantic coast of North America.
- Active Continental Margins:
- Definition: Located at convergent plate boundaries where oceanic lithosphere is being subducted beneath the leading edge of a continent.
- Major Features: Deep-ocean trenches, accretionary wedges, and subduction erosion features.
- Location: Typically found bordering the Pacific Ocean, where subduction zones are prevalent.
- Passive Continental Margins:
- How are active continental margins related to plate tectonics?
- Active continental margins are closely related to plate tectonics, specifically the process of subduction. At these margins, oceanic lithosphere is being subducted beneath continental plates, leading to tectonic activity such as the formation of deep-ocean trenches, accretionary wedges, and subduction erosion features. The movement and interaction of tectonic plates drive the geological processes observed at active continental margins, highlighting the connection between plate tectonics and the Earth's surface features.
13.3 Features of Deep-Ocean Basins p.371
1. Major Features of Deep-Ocean Basins:
- Deep-Ocean Trenches:
- Long, relatively narrow troughs, often the deepest parts of the ocean.
- Typically found along the margins of the Pacific Ocean.
- Example: Challenger Deep in the Mariana Trench.
- Abyssal Plains:
- Deep, incredibly flat features, the most level places on Earth.
- Covered by thick accumulations of sediment, primarily consisting of fine sediments from land, mineral matter precipitated out of seawater, and shells/skeletons of marine organisms.
- Volcanic Structures:
- Seamounts and Volcanic Islands:
- Submarine volcanoes rising hundreds of meters above the surrounding seafloor.
- Some grow large enough to become oceanic islands.
- Guyots:
- Submerged, flat-topped seamounts formed by inactive volcanic islands.
- Oceanic Plateaus:
- Massive structures resembling lava plateaus composed of flood basalts.
- Generated from vast outpourings of fluid basaltic lavas.
- Seamounts and Volcanic Islands:
2. Darwin's Hypothesis on Coral Atolls:
- Charles Darwin's hypothesis explains the origin of ring-shaped coral atolls.
- Darwin proposed that volcanic islands gradually sink over time, and corals respond by building the reef complex upward.
- Atolls may eventually become guyots when the rate of subsidence exceeds the ability of corals to build up the reef or when reef-building organisms die out.
Key Points:
- Deep-Ocean Trenches: Formed at plate boundaries where oceanic lithosphere subducts, associated with volcanic activity and earthquake generation.
- Abyssal Plains: Covered by thick accumulations of sediment, primarily composed of fine sediments, mineral matter, and marine organisms' remains.
- Volcanic Structures: Include seamounts, volcanic islands, guyots, and oceanic plateaus, formed by underwater volcanic activity and processes.
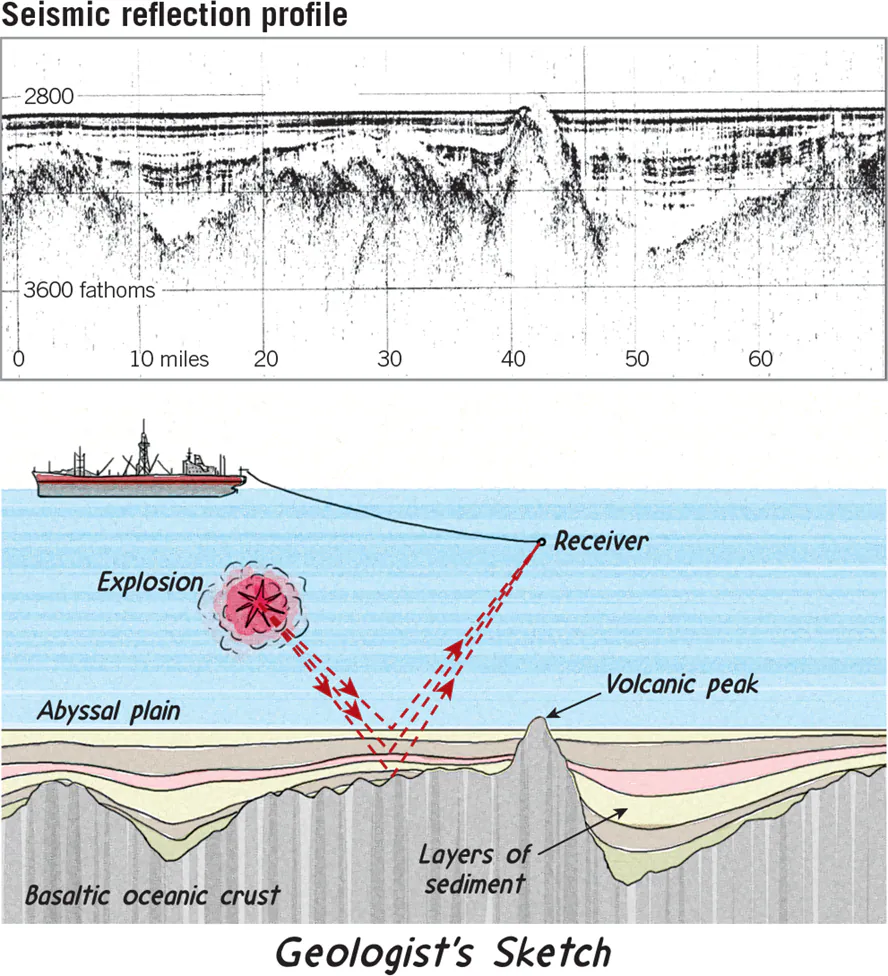
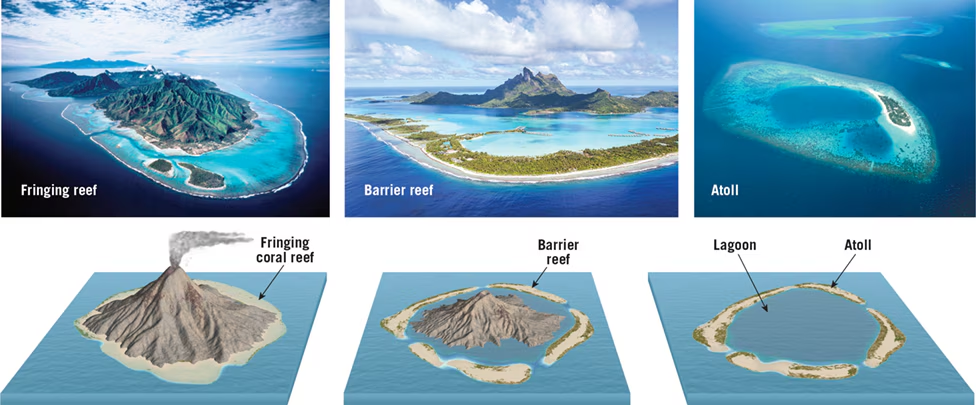
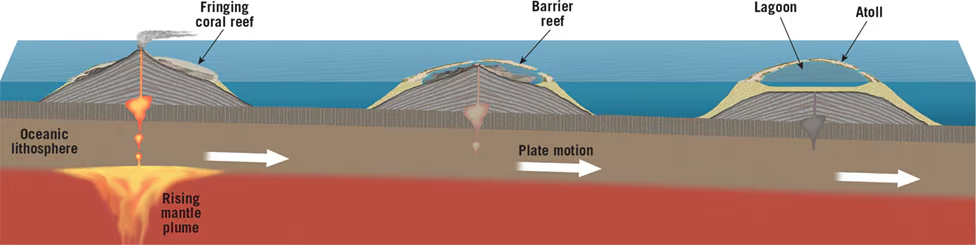
13.3 Review Questions
1. Explain how deep-ocean trenches are related to convergent plate boundaries.
- Answer: Deep-ocean trenches are formed at convergent plate boundaries where oceanic lithosphere is being subducted beneath the leading edge of a continent or another oceanic plate. Trenches are sites of plate convergence where slabs of oceanic lithosphere subduct and plunge back into the mantle. This process of subduction leads to the formation of deep, narrow furrows known as deep-ocean trenches.
2. Why are abyssal plains more extensive on the floor of the Atlantic than on the floor of the Pacific?
- Answer: Abyssal plains are more extensive on the floor of the Atlantic than on the floor of the Pacific because the Atlantic Ocean has fewer trenches to act as traps for sediment carried down the continental slope. Abyssal plains owe their relatively featureless topography to thick accumulations of sediment that have buried an otherwise rugged ocean floor. With fewer trenches in the Atlantic to trap sediment, the accumulation of sediment is more extensive, resulting in more extensive abyssal plains.
3. How does a flat-topped seamount, called a guyot, form?
- Answer: Flat-topped seamounts, known as guyots, form when inactive volcanic islands gradually sink and disappear below the water surface. During their existence, inactive volcanic islands are gradually lowered to near sea level by weathering and erosion. As a moving plate carries inactive volcanic islands away from the elevated oceanic ridge or hot spot over which they formed, they gradually sink. Submerged, flat-topped seamounts that formed in this manner are called guyots.
4. Using Darwin’s hypothesis, place these coral reefs in order from youngest to oldest: barrier reef, atoll, and fringing reef.
- Answer: According to Darwin's hypothesis, coral reefs progress in the following order from youngest to oldest:
- Fringing Reef: Forms along the margins of a volcano.
- Barrier Reef: Develops with a volcano in the middle, creating a lagoon between the reef and the shore.
- Atoll: Consists of a continuous or broken ring of coral reef surrounding a central lagoon, indicating the sinking of the volcano beneath the sea level and subsequent reef growth.
Chapter 18
18.1 Ocean Waves and Shoreline Dynamics
1. Deep-Ocean Trenches and Convergent Plate Boundaries
- Definition: Deep-ocean trenches are long, narrow furrows formed at convergent plate boundaries where oceanic lithosphere is being subducted beneath another plate.
- Details: Trenches are sites of plate convergence where oceanic plates plunge back into the mantle, generating deep depressions in the ocean floor.
2. Abyssal Plains and Oceanic Floors
- Definition: Abyssal plains are extensive, flat regions on the ocean floor.
- Details: They are more extensive in the Atlantic Ocean due to fewer trenches, allowing sediment accumulation. Sediment accumulation buries rugged ocean floor, creating flat plains.
3. Formation of Guyots
- Definition: Guyots are flat-topped seamounts formed from inactive volcanic islands.
- Details: Inactive volcanic islands gradually sink below sea level due to erosion and plate motion, resulting in submerged flat-topped seamounts known as guyots.
4. Coral Reef Development According to Darwin's Hypothesis
- Definition: Coral reefs include fringing reefs, barrier reefs, and atolls.
- Details: According to Darwin, coral reefs progress from fringing reefs (youngest), forming along volcanic margins, to barrier reefs (middle-aged), with a central lagoon, and finally atolls (oldest), representing submerged volcanic islands with surrounding coral reefs.
5. Shoreline Dynamics
- Definition: The shoreline is the dynamic interface where land and sea meet.
- Details: It undergoes constant modification by waves, erosion, and sediment transport, exhibiting a complex character influenced by various geologic processes.
6. Ocean Wave Characteristics
- Definition: Ocean waves are generated by wind and carry energy across the sea surface.
- Details: Wave characteristics include wave height, wavelength, and wave period, influenced by wind speed, duration, and fetch. Waves transform into swells and travel great distances across ocean basins.
7. Circular Orbital Motion of Waves
- Definition: Circular orbital motion describes the movement of water particles within waves.
- Details: Water particles move in circular orbits as waves travel forward, with diminishing motion with depth. Circular motion allows energy transfer through water without significant forward movement.
8. Wave Behavior Near Shore
- Definition: Wave behavior changes as waves approach the shore.
- Details: As waves encounter shallow water, their speed decreases, wavelength shortens, and wave height increases. Eventually, waves break, forming surf, and water rushes up the shore as swash, then back down as backwash.
When water depth is less than half the wavelength, wave speed decreases, and the faster-moving waves farther from shore begin to catch up, causing the distance between waves (the wavelength) to decrease. This causes an increase in wave height, to the point where the waves finally pitch forward and break in the surf zone. The first portion of the animation illustrates the ideas presented in this figure.
20.1 Question Review p.563
1. Why is the shoreline described as being an interface?
Answer: The shoreline is described as an interface because it marks the dynamic boundary where land, air, and sea interact. It's a zone where the ocean's water meets the land, resulting in constant changes and interactions between these different elements.
2. List three factors that determine the height, length, and period of a wave.
Answer:
- Wind Speed: Faster winds generate larger waves.
- Duration of Wind: Longer durations result in larger waves.
- Fetch: The distance over which the wind blows across the water's surface determines wave size.
3. Describe the motion of a floating object as a wave passes.
Answer: As a wave passes, a floating object moves in a circular orbital motion. It rises as the crest approaches, moves forward as the crest passes, descends as the trough approaches, and moves backward as the trough passes. This movement results in a circular path for the floating object.
4. How do a wave’s speed, wavelength, and height change as the wave moves into shallow water and breaks?
Answer:
- Wave Speed: Wave speed decreases as the wave moves into shallow water due to interaction with the seafloor.
- Wavelength: Wavelength shortens as the wave approaches the shore, causing waves to become closer together.
- Wave Height: Wave height increases as the wave enters shallow water and eventually breaks. Breaking waves result in higher wave heights due to the compression of energy as the wave front collapses.
20.2 Beaches and Shoreline Processes
Definitions:
- Beach: An accumulation of sediment found along the landward margin of a water body, composed of locally abundant materials such as sand, gravel, or shell fragments.
- Wave Erosion: The process by which waves impact, pressure, and abrasion erode the shoreline, cliffs, and other coastal features.
- Wave Refraction: The bending of waves as they approach the shore, affecting the distribution of energy along the shoreline and influencing erosion, sediment transport, and deposition.
Important Details:
- Wave Erosion and Sediment Movement:
- Waves erode the shoreline through impact, pressure, and abrasion during storms.
- Abrasion is intensified in the surf zone, leading to the smoothing and rounding of rocks along the shore.
- Sediment on the beach is in constant motion due to wave action, leading to the transport of sand and other materials along the shoreline.
- Sand Movement on the Beach:
- Wave energy moves sand along the beach face and in the surf zone, both parallel and perpendicular to the shoreline.
- Swash and backwash movements determine the direction of sand movement on the beach.
- Beaches experience seasonal changes in width due to variations in wave energy, with wide beaches forming during calm periods and narrower beaches during stormy periods.
- Wave Refraction:
- Wave refraction causes waves to bend as they approach shallow water and align nearly parallel to the shoreline.
- Differential wave attack along irregular coastlines results in erosion of headlands and sediment deposition in bays, leading to shoreline straightening over time.
- Longshore Transport:
- Beach drift and longshore currents transport sediment in a zigzag pattern along the beach face.
- Longshore currents move substantially more sediment than beach drift and can transport large quantities of sand and gravel along the shore.
- Longshore currents flow southward along both the Atlantic and Pacific shores of the United States.
- Rip Currents:
- Rip currents are concentrated movements of water flowing opposite to breaking waves, often recognized by their interference with incoming waves.
- Rip currents can be hazardous to swimmers, carrying them away from the shore, and the best strategy for exiting a rip current is to swim parallel to the shore for a few tens of meters.
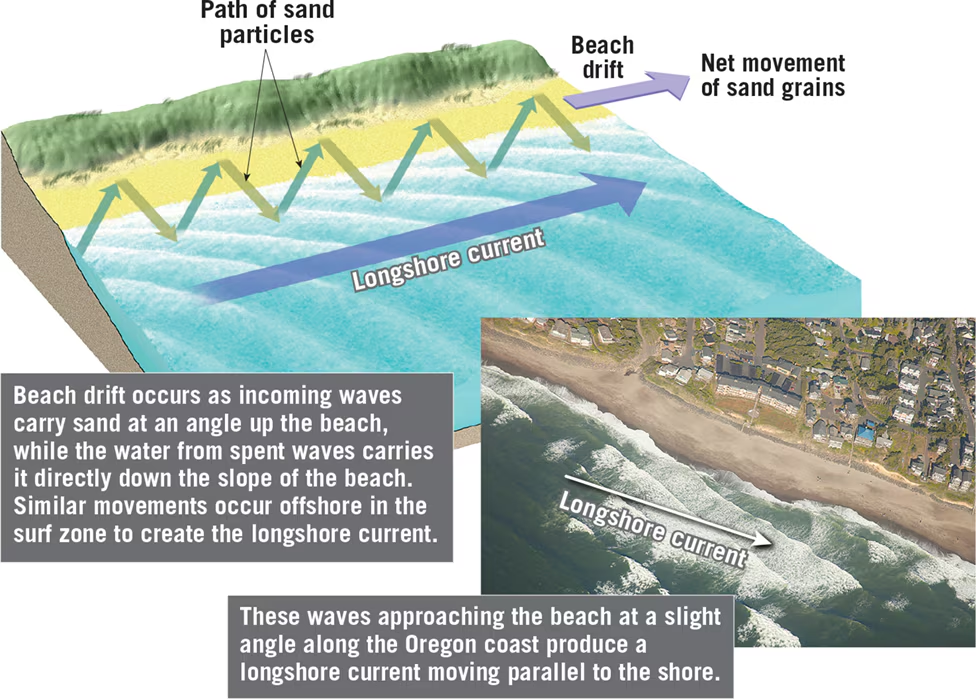
20.2 Review Questions
- Why do waves approaching the shoreline often bend?
Waves approaching the shoreline often bend due to wave refraction. This bending occurs because the part of the wave nearest the shore reaches shallow water and slows first, whereas the end that is still in deep water continues forward at its full speed. As a result, the wave front may approach nearly parallel to the shore, regardless of the original direction of the wave.
- What is the effect of wave refraction along an irregular coastline?
Wave refraction along an irregular coastline affects the distribution of wave energy along the shore, leading to differential wave attack. Headlands that project into the water experience concentrated wave impact from waves bending around them, causing erosion. In contrast, bays experience weakened wave activity due to wave divergence, leading to sediment accumulation and beach formation. Over time, wave refraction can contribute to shoreline straightening as erosion occurs in headlands and sediment deposition occurs in bays.
- Describe the two processes that contribute to longshore transport.
Longshore transport involves two main processes:
- Beach Drift: Beach drift occurs when the uprush of water from breaking waves (swash) moves sediment obliquely along the beach face. This process transports sand and pebbles parallel to the shoreline, with the swash carrying sediment up the beach face and the backwash returning sediment down the beach slope.
- Longshore Currents: Longshore currents are generated by waves approaching the beach at an angle. These currents flow parallel to the shoreline within the surf zone and move substantially more sediment than beach drift. Longshore currents transport fine suspended sand and roll larger sand and gravel along the bottom, contributing to the movement of sediment along the shore.
20.3 Shoreline Features
Definitions:
- Erosional Features: Landforms along coastlines formed primarily by erosional processes.
- Depositional Features: Coastal features created by the deposition of sediment transported by various processes.
Important Details:
- Erosional Features:
- Wave-Cut Cliffs: Formed by the cutting action of surf against the base of coastal land, leading to the retreat of the cliff.
- Wave-Cut Platforms: Flat, benchlike surfaces left behind by the receding cliff as erosion progresses.
- Marine Terraces: Wave-cut platforms uplifted above sea level by tectonic forces, recognized by gentle seaward-sloping shape.
- Sea Arches and Sea Stacks: Result from vigorous wave attack on headlands, with sea arches forming initially and sea stacks as isolated remnants.
- Depositional Features:
- Spits: Elongated ridges of sand projecting from the land into the mouth of an adjacent bay, often with a hooked end.
- Baymouth Bars: Sandbars completely crossing a bay, sealing it off from the open ocean.
- Tombolos: Sand ridges connecting an island to the mainland or to another island, formed similarly to spits.
- Barrier Islands: Low ridges of land parallel to the coast, located offshore and separated from the mainland by lagoons.
- Barrier Islands:
- Formed through various processes, including spits severed from the mainland, accumulation of sand from turbulent waters, and former sand dune ridges flooded by rising sea levels.
- Characterized by sand dunes, lagoons, and coastal marshes, providing protection to the mainland from storm waves.
- The Evolving Shore:
- Shorelines undergo continuous modification due to erosional and depositional processes.
- Irregular coastlines may become straighter and more regular over time due to marine erosion and deposition.
- Features such as cliffs, platforms, spits, and bars illustrate the evolutionary process of coastal landforms.
20.3 Review Questions
- How is a marine terrace related to a wave-cut platform?
- Answer: A marine terrace is related to a wave-cut platform as it is essentially an uplifted wave-cut platform. Both features are formed by marine erosion along coastlines. While a wave-cut platform is a flat, benchlike surface left behind by the receding cliff due to wave erosion, a marine terrace is a wave-cut platform that has been uplifted above sea level by tectonic forces.
- Describe the formation of each labeled feature in Figure 20.17.
- Answer:
- A: Initially irregular coast with headlands and bays.
- B: Formation of wave-cut cliffs due to erosion by pounding surf.
- C: Retreat of cliffs creates wave-cut platforms.
- D: Deposition of sediment in bays and formation of spits.
- E: River sediment fills bays while spits grow, forming baymouth bars.
- F: Final stage with a generally straight, smooth coast resulting from erosion and deposition processes.
- Answer:
- List three ways that a barrier island may form.
- Answer:
- Barrier islands may form from spits that were severed from the mainland by wave erosion or rising sea levels.
- They can also be created when turbulent waters heap up sand scoured from the ocean bottom along the line of breakers.
- Barrier islands may originate as former sand dune ridges along the shore during periods of lower sea levels, which were flooded as sea levels rose.
- Answer:
20.4 Contrasting American Coastlines
Definitions:
- Emergent Coasts: Coastal areas that experience either uplift or a drop in sea level, leading to the exposure of wave-cut cliffs and platforms above sea level.
- Submergent Coasts: Coastal areas that show signs of submergence due to rising sea levels or land subsidence, resulting in irregular coastlines and flooded river valleys known as estuaries.
Important Facts:
- Emergent Coasts:
- Examples include portions of coastal California and regions once buried beneath ice sheets.
- In California, uplift has resulted in the formation of marine terraces, such as the Palos Verdes Hills.
- Areas like the Hudson Bay region of Canada are still experiencing uplift, with rates exceeding 1 centimeter per year.
- Submergent Coasts:
- Characterized by irregular coastlines and estuaries formed by flooded river valleys.
- Examples include Chesapeake and Delaware Bays along the Atlantic coastline and the coast of Maine.
- Irregularities in submergent coasts are caused by the sea flooding lower river valleys while leaving ridges projecting as headlands.
- Erosion Problems on Atlantic and Gulf Coasts:
- Barrier islands, common along these coasts, face erosion issues due to their exposure to major storms.
- Storm waves cause movement of sand, erosion of dunes, and overwash, threatening coastal developments.
- Attempts to protect developments from erosion often lead to exacerbation of the problem.
- Erosion Problems on Pacific Coast:
- Narrow beaches backed by steep cliffs characterize much of the Pacific coast.
- Interruption of natural sand supply by dams for irrigation and flood control leads to beach narrowing.
- Narrowed beaches result in accelerated erosion of sea cliffs during storm events.
- Urbanization and development on cliffs contribute to erosion through runoff and destabilization.
- Coastal Vulnerability:
- Sporadic occurrence of storms leads to variable shoreline erosion along the Pacific coast.
- Damage from erosion is often attributed to storms rather than human interventions like coastal development and sediment-trapping dams.
- With rising sea levels, increased erosion and sea-cliff retreat are expected along many parts of the Pacific coast in the future.
2.4 Review Questions
- Are estuaries associated with submergent or emergent coasts? Explain.
- Estuaries are associated with submergent coasts. These coastal features are formed when sea levels rise or the land adjacent to the sea subsides. Estuaries occur when lower river valleys are flooded by the rising sea, leaving ridges projecting as headlands. This process characterizes submergent coasts, where the coastline shows signs of being submerged due to rising sea levels.
- What observable features would lead you to classify a coastal area as emergent?
- Observable features indicating an emergent coast include:
- Exposed wave-cut cliffs and platforms above sea level.
- Marine terraces formed by the uplift of former wave-cut platforms.
- Terraces at various elevations indicating multiple episodes of uplift.
- Ancient shorelines and coastal features high above current sea levels.
- These features suggest that the coastal area has experienced uplift or a drop in sea level, leading to the exposure of previously submerged landforms.
- Observable features indicating an emergent coast include:
- How might building a dam on a river that flows to the sea affect a coastal beach?
- Building a dam on a river that flows to the sea can have several effects on a coastal beach:
- Reduction of Sediment Supply: Dams trap sediment that would naturally flow downstream to replenish coastal beaches. This reduction in sediment supply can lead to beach erosion and narrowing.
- Altered River Mouth Dynamics: Dams can modify the natural flow of rivers, impacting sediment deposition at river mouths. Reduced sediment transport to the coast can result in diminished sand deposition on beaches, affecting their stability.
- Increased Coastal Vulnerability: With reduced sediment supply, coastal beaches may become more vulnerable to erosion from wave action and storm events. Narrowed beaches offer less protection to coastal infrastructure and can lead to increased property damage during storms.
- Overall, building a dam on a river can disrupt the natural processes that maintain coastal beaches, resulting in erosion and loss of coastal habitat.
- Building a dam on a river that flows to the sea can have several effects on a coastal beach:
20.5 p.580 Track Forecasts
Study Guide: Track Forecasts
1. Definition:
- Track Forecast: The predicted path of a hurricane or tropical cyclone. It is crucial for anticipating the trajectory of the storm and making informed decisions regarding evacuation and preparedness measures.
2. Importance:
- Critical Information: Track forecasts provide essential information for assessing the potential impact of a hurricane on specific regions, allowing authorities to issue timely warnings and evacuation orders.
- Evacuation Planning: Accurate track forecasts enable the identification of areas at risk of storm surge and other hazards, facilitating efficient evacuation planning and minimizing loss of life.
- Improving Accuracy: Advances in technology and forecasting techniques have led to significant improvements in track forecast accuracy over the years, enhancing preparedness and response efforts.
3. Historical Trends:
- Improving Accuracy: Over the past two decades, track forecast accuracy has steadily improved. Current 5-day track forecasts are as accurate as the 3-day forecasts from 20 years ago, indicating substantial progress in hurricane prediction capabilities.
- Forecast Uncertainty: Despite improvements, forecast uncertainty still necessitates issuing hurricane warnings for relatively large coastal areas. Approximately one-quarter of the warning area typically experiences hurricane conditions.
4. Forecast Cone:
- Definition: When the National Hurricane Center issues a hurricane track forecast, it is represented as a "forecast cone."
- Probable Track: The cone depicts the probable path of the storm's center, enclosing the area swept out by a series of circles along the forecast track (e.g., at 12 hours, 24 hours, 36 hours, etc.).
- Prediction Confidence: The circles within the cone expand as the forecast extends into the future, indicating increasing uncertainty. The entire track of the storm is expected to remain within the cone approximately 60 to 70 percent of the time.
5. Practical Implications:
- Public Awareness: Understanding the forecast cone and its implications helps the public and emergency responders prepare for potential hurricane impacts, including storm surge, high winds, and heavy rainfall.
- Emergency Management: Emergency management agencies use track forecasts to allocate resources, implement evacuation plans, and coordinate response efforts, enhancing community resilience and disaster preparedness.
6. Ongoing Advancements:
- Technological Innovation: Ongoing advancements in satellite imagery, computer modeling, and data assimilation techniques continue to enhance the accuracy and reliability of hurricane track forecasts.
- Collaborative Efforts: International collaboration among meteorological agencies and research institutions contributes to the development of more sophisticated forecasting models and tools, further improving track forecast accuracy.
Key Takeaway:
Accurate track forecasts are essential for informing evacuation decisions, mitigating risks, and reducing the impact of hurricanes on coastal communities. While significant progress has been made in forecast accuracy, ongoing efforts are needed to further enhance predictive capabilities and ensure effective disaster preparedness and response.
20.6 Stabilizing the Shore
1. Overview:
- Shoreline Erosion: Shoreline erosion is a dynamic process that poses significant challenges to coastal communities. Various methods are employed to address erosion problems and protect coastal property.
2. Hard Stabilization:
- Definition: Hard stabilization involves constructing structures to protect the coast from erosion and prevent sand movement.
- Examples: Jetties, groins, breakwaters, and seawalls are common forms of hard stabilization.
- Drawbacks: Hard stabilization can disrupt natural processes, cause unintended consequences such as beach erosion downstream, and require ongoing maintenance.
3. Jetties:
- Purpose: Jetties are built at the entrances of rivers and harbors to prevent sediment deposition in navigation channels.
- Impact: Jetties can impede natural sand movement, leading to beach erosion on one side while causing sediment buildup on the other.
4. Groins:
- Function: Groins are barriers constructed perpendicular to the shoreline to trap sand moving parallel to the coast.
- Issues: Groins can disrupt natural sediment transport, leading to erosion downstream and the proliferation of groin fields.
5. Breakwaters and Seawalls:
- Description: Breakwaters protect boats by creating a quiet water zone near the shoreline, while seawalls armor the coast against wave action.
- Consequences: Breakwaters can disrupt sand movement and lead to beach filling, while seawalls accelerate erosion by reflecting wave energy seaward.
6. Alternatives to Hard Stabilization:
- Beach Nourishment: Involves adding sand to beaches to widen the shoreline and mitigate erosion, but it is costly and temporary.
- Changing Land Use: Involves relocating or abandoning vulnerable structures in high-hazard coastal areas to allow natural beach processes to occur.
7. Practical Examples:
- New York's Staten Island: After Hurricane Sandy, vulnerable shoreline areas were transformed into waterfront parks to protect inland properties and provide recreational space.
- Policy Shift: Some coastal communities are reconsidering land-use policies to prioritize safety and resilience over coastal development.
8. Considerations:
- Cost vs. Benefit: Evaluating the effectiveness and sustainability of shoreline stabilization methods requires consideration of costs, environmental impacts, and long-term benefits.
- Community Engagement: Coastal management decisions should involve input from stakeholders, policymakers, scientists, and engineers to balance competing interests and ensure sustainable coastal development.
Key Takeaway:
Addressing shoreline erosion requires a multifaceted approach that considers both natural processes and human activities. While hard stabilization techniques offer immediate protection, alternatives such as beach nourishment and changing land use policies prioritize long-term resilience and environmental sustainability. Coastal management strategies should strive for a balance between protecting property and preserving natural coastal ecosystems.
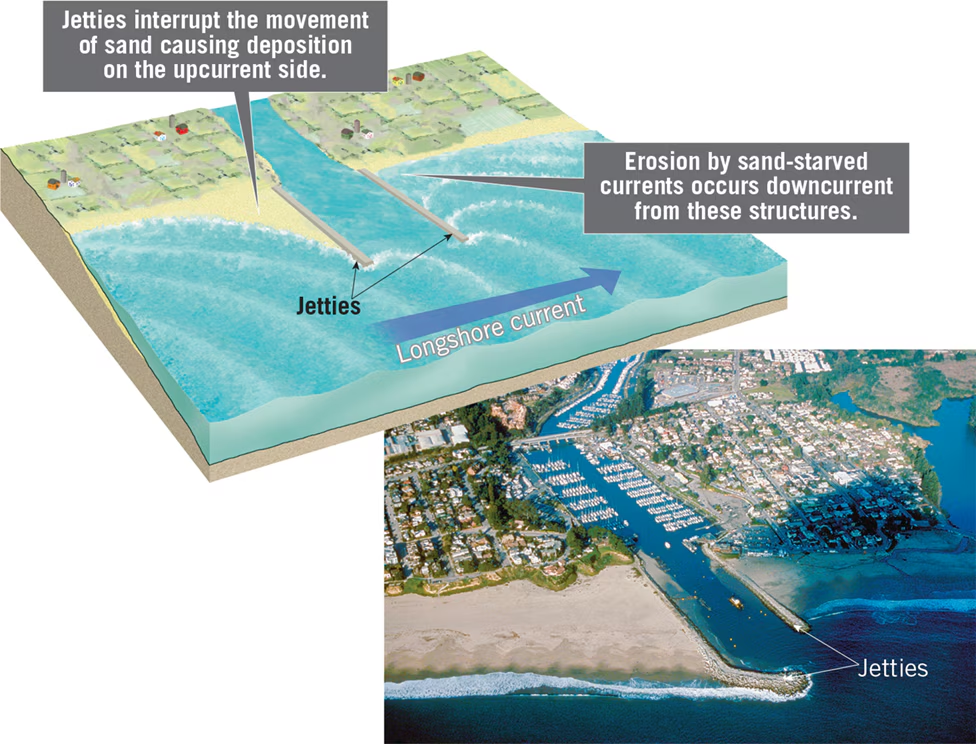
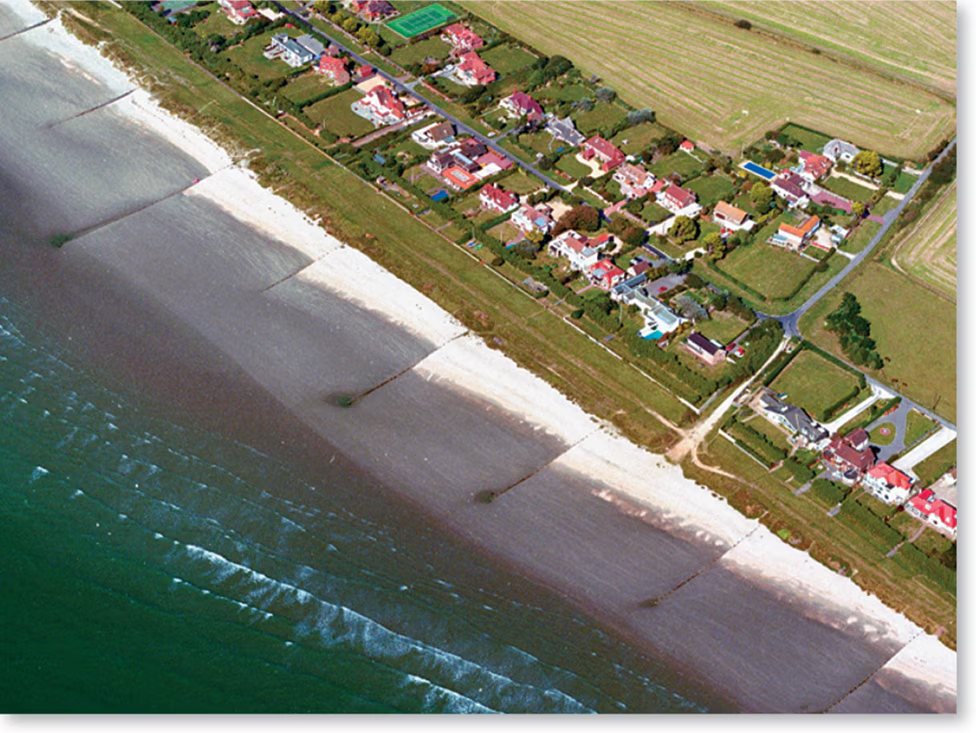
Groins
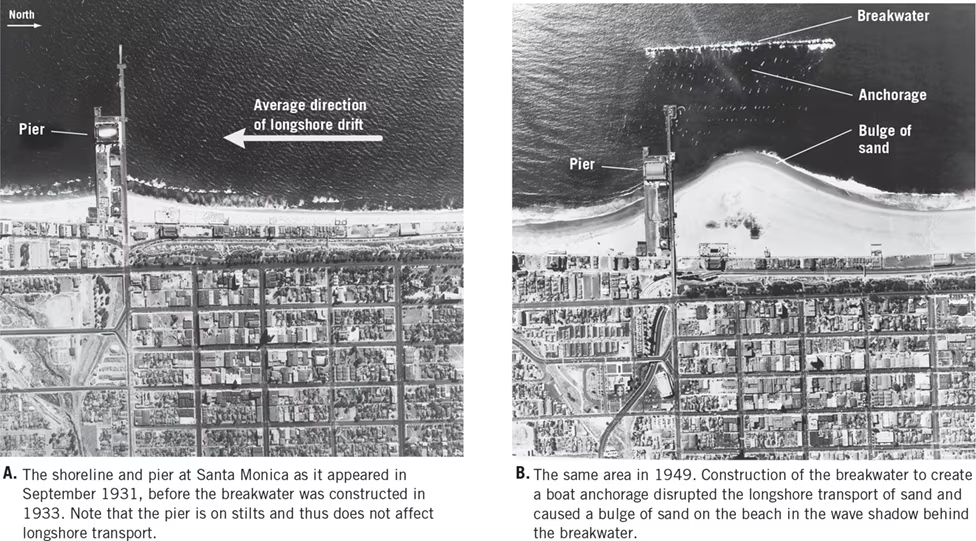
20.6 Review Questions
1. List at least three examples of hard stabilization and describe what each is intended to do:
a) Jetties:
- Intended Purpose: Jetties are built at the entrances of rivers and harbors to prevent sediment deposition in navigation channels.
- Function: They help maintain navigation channels by confining water flow to a narrow zone, preventing sediment accumulation and ensuring safe passage for ships.
- Impact on Sand Distribution: Jetties can disrupt natural sand movement, causing erosion on one side where sediment accumulates and deposition on the other side where sediment is trapped.
b) Groins:
- Intended Purpose: Groins are barriers constructed perpendicular to the shoreline to trap sand moving parallel to the coast.
- Function: They are designed to retain sediment and widen beaches by interrupting the longshore drift of sand along the coastline.
- Impact on Sand Distribution: Groins can disrupt natural sediment transport, leading to erosion downstream of the structure and the formation of groin fields, where sediment accumulates updrift.
c) Seawalls:
- Intended Purpose: Seawalls are vertical or sloping structures built parallel to the shoreline to armor the coast against wave action.
- Function: They protect coastal property from erosion by reflecting wave energy seaward and reducing the impact of waves on the shore.
- Impact on Sand Distribution: Seawalls can accelerate erosion by preventing natural sand replenishment from the sea, leading to the narrowing or disappearance of beaches in front of the wall.
2. What are two alternatives to hard stabilization, and what are the potential problems associated with each?
a) Beach Nourishment:
- Alternative Method: Beach nourishment involves adding sand to beaches to widen the shoreline and mitigate erosion.
- Potential Problems:
- Costly: Beach nourishment projects require significant financial investment for the acquisition and transportation of sand.
- Temporary Solution: The replenished sand is subject to the same erosional forces as the original beach, requiring periodic replenishment.
- Environmental Impact: Sourcing sand from offshore areas or inland quarries can disrupt ecosystems and habitats.
b) Changing Land Use:
- Alternative Method: Changing land use involves relocating or abandoning vulnerable structures in high-hazard coastal areas to allow natural beach processes to occur.
- Potential Problems:
- Social Resistance: Residents and property owners may resist relocating or abandoning coastal properties due to sentimental attachment or financial interests.
- Economic Impacts: Relocating infrastructure and properties away from the coast can result in economic losses for individuals and communities reliant on coastal tourism and development.
- Governance Challenges: Implementing land-use policies that prioritize safety and resilience over coastal development requires coordination among various stakeholders and regulatory bodies.
20.7 Tides
1. Causes of Tides:
- Tides are the daily changes in the elevation of the ocean surface caused by the gravitational interactions of Earth with the Moon and Sun.
- Sir Isaac Newton explained that ocean tides result from the gravitational attraction exerted upon Earth by the Moon and, to a lesser extent, by the Sun.
- The gravitational force of the Moon causes two tidal bulges on Earth's surface, one on the side facing the Moon and the other on the opposite side.
2. Monthly Tidal Cycle:
- The primary influence on tides is the Moon, which makes one complete revolution around Earth every 29.5 days.
- Near the times of new and full moons, the combined gravitational forces of the Moon and Sun cause larger tidal bulges, resulting in spring tides.
- At first and third quarters of the Moon, the gravitational forces of the Moon and Sun act at right angles, resulting in smaller tidal ranges known as neap tides.
- Each month experiences two spring tides and two neap tides, occurring approximately one week apart.
3. Tidal Patterns:
- Tidal patterns are influenced by various factors, including the shape of the coastline, ocean basin configuration, and water depth.
Three main tidal patterns exist worldwide:
a) Diurnal Tides: Characterized by one high tide and one low tide each tidal day.
b) Semidiurnal Tides: Exhibit two high tides and two low tides of approximately equal heights each tidal day.
- c) Mixed Tides: Similar to semidiurnal tides but with a large inequality in high and low water heights, occurring each tidal day.
4. Tidal Currents:
- Tidal currents are horizontal flows of water that accompany the rise and fall of the tide, induced by tidal forces.
- Flood currents move into coastal zones as the tide rises, while ebb currents move seaward as the tide falls.
- Slack water refers to periods of little or no current between flood and ebb.
- Tidal currents can be rapid in narrow areas such as bays, estuaries, and straits, where they may attain high speeds.
- Tidal currents are not generally major agents of erosion and sediment transport, but they can create deposits called tidal deltas in certain areas, particularly around inlets.
Key Concepts:
- Tides result from gravitational interactions between Earth, the Moon, and the Sun.
- Monthly tidal cycles include spring tides (large tidal ranges) and neap tides (small tidal ranges).
- Tidal patterns vary globally, with diurnal, semidiurnal, and mixed tides.
- Tidal currents accompany the rise and fall of tides and can influence coastal dynamics, including erosion and sediment transport.
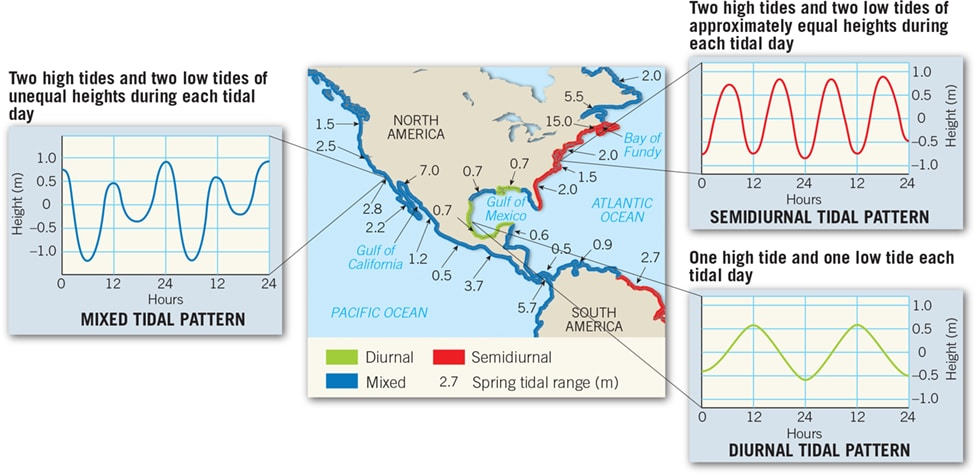
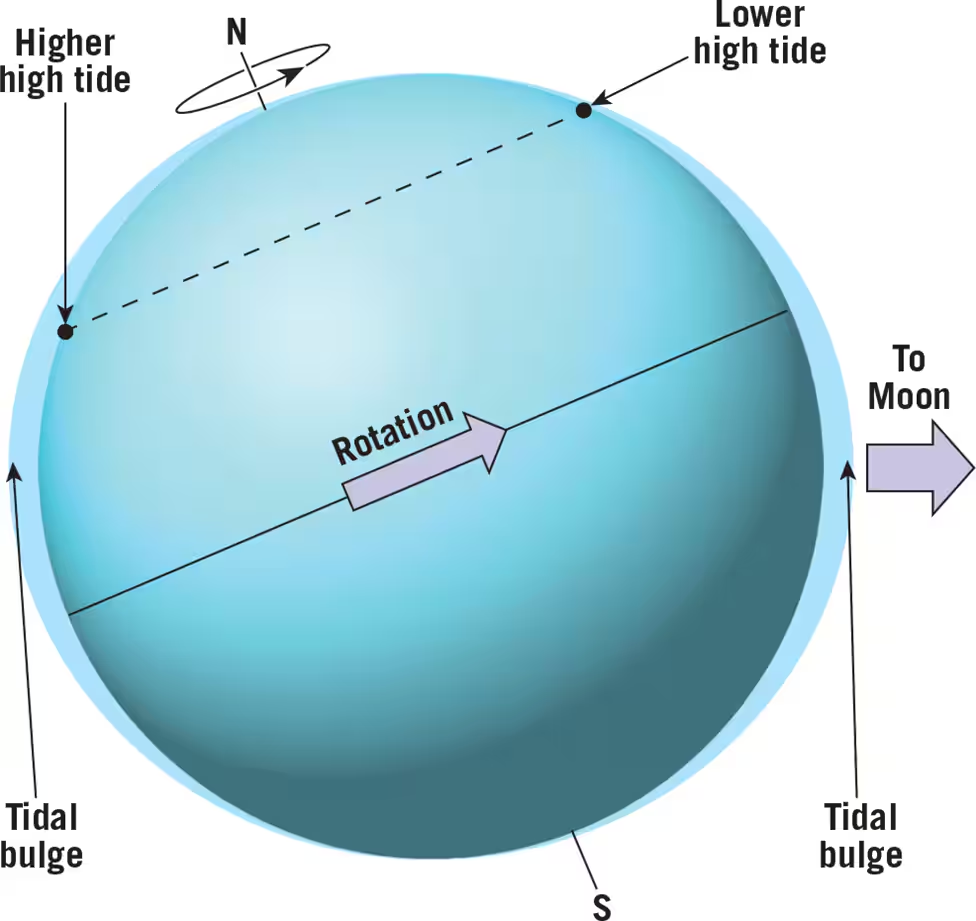
If Earth were covered to a uniform depth with water, there would be two tidal bulges: one on the side of Earth facing the Moon (right) and the other on the opposite side of Earth (left). Depending on the Moon’s position, tidal bulges may be inclined relative to Earth’s equator. In this situation, Earth’s rotation causes an observer to experience two unequal high tides during a day.
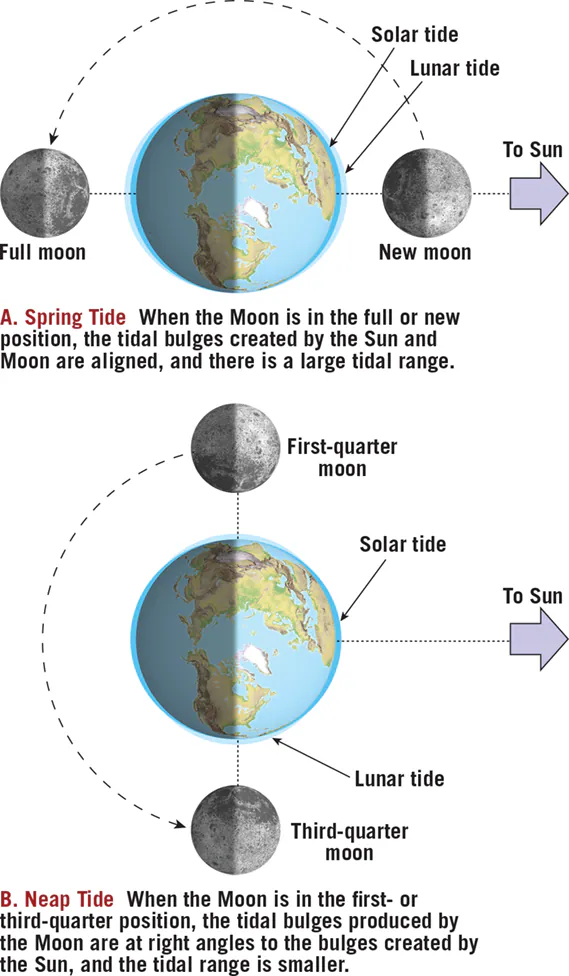
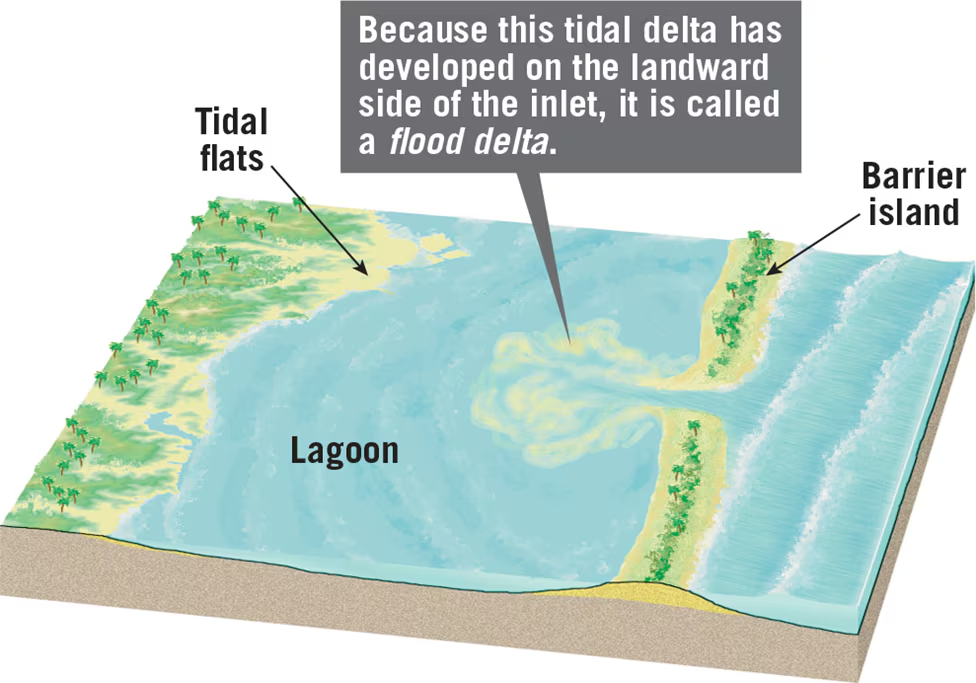
20.7 Question Review
Explain why an observer can experience two unequal high tides during a single day:
- An observer can experience two unequal high tides during a single day due to the inclination of the tidal bulges relative to Earth's equator. Depending on the Moon's position, the tidal bulges may be inclined, resulting in variations in tidal heights. When the tidal bulge is inclined, the gravitational forces acting on the water are not balanced, leading to unequal high tides. As Earth rotates, an observer may pass through areas where the tidal bulge is more pronounced, resulting in a higher high tide, and areas where it is less pronounced, leading to a lower high tide. Therefore, the observer experiences two unequal high tides within a single day.
- Distinguish between neap tides and ebb tides:
- Neap tides occur when the gravitational forces of the Moon and Sun act on Earth at right angles, partially offsetting each other's influence. As a result, the tidal range is smaller during neap tides compared to spring tides. Neap tides occur approximately during the first and third quarters of the Moon.
- Ebb tides, on the other hand, refer to the period of tidal current flow when seaward-moving water generates ebb currents. These currents occur as the tide falls and the water moves away from the coast. Ebb tides are a specific phase of the tidal cycle and are characterized by the movement of water from coastal areas towards the open ocean.
- Contrast flood current and ebb current:
- Flood current refers to the period of tidal current flow when water moves into coastal zones as the tide rises. This movement of water towards the coast is driven by the rising tide and is known as flood current. Flood currents typically occur during the first half of the tidal cycle.
- Ebb current, on the other hand, occurs during the period of tidal current flow when water moves seaward as the tide falls. As the tide ebbs, water flows away from the coast towards the open ocean, generating ebb currents. Ebb currents are characteristic of the second half of the tidal cycle and represent the outward movement of water from coastal areas.
Practice Questions:
3.) Geologists have discovered long, generally straight chains of volcanic islands within ocean basins that get progressively younger or progressively older depending on the direction of travel along the volcanic chain. The Hawaiian Islands are an example of such a chain. What is the origin of such a volcanic chain?
a.) magma intruding along the boundary between two lithospheric plates.
b.) magma intruding from a large region of flood basalts.
c.) magma intruding within an island arc.
d.) magma intruding along a mid-ocean fracture zone.
e.) magma intruding from a hotspot within the mantle.
39.) Suppose that you discovered coal deposits over glacial tills in rocks at the northern tip of Greenland. How would you interpret this discovery.
a.) The earth's cool conditions once favored rapid planet growth at the latitude of northern Greenland.
b.) You conclude that you made a mistake in identifying the till because coal can not possibly be deposited on top of glacial tills.
c.) The continent of Greenland must have drifted from a polar latitude to a tropical latitude and back again.
d.) The coal was deposited by the present continental glaciers covering the Greenland ice cap.
e.) You make a note of this outcrop but you are not excited because coal and till are commonly found in the same outcrop.
46.) Recall the block diagrams of faults in your text book. What is the name of the fault which has a hanging wall moving downward relative to the foot wall?
a.) a reverse fault.
b.) a normal fault.
c.) a strike-slip fault.
d.) a transform fault.
e.) a thrust fault.
55.) The following two locations are examples of hot spots:
a.) State College, PA and Washington D. C.
b.) Hawaii and the East Pacific Rise.
c.) Hawaii and Yellowstone.
d.) Mid Atlantic Ridge and Cascades.
e.) Sumatra and Hawaii.
64.) Which of the following geological periods is oldest?
a.) Cretaceous.
b.) Tertiary.
c.) Jurassic
d.) Cambrian.
e.) Devonian.
98.) Of the three types of seismic waves, which cause the most damage during an earthquake?
a.) compressional waves.
b.) shear waves.
c.) transverse waves.
d.) longitudinal waves.
e.) surface waves.
Geology Final Water
Chapter 9:
P.264-284
Relative Dating Principles
Numerical vs Relative Dates
- Numerical dates specify the actual number of years that have passed since an event occurred, determined through radiometric dating techniques.1
- Relative dates establish the sequence of events (which came first, second, third, etc.) without providing numerical ages. Relative dating principles were developed before radiometric dating methods.1
Principle of Superposition
- In an undeformed sequence of sedimentary rocks, each bed is older than the one above and younger than the one below.1
Principle of Original Horizontality
- Sedimentary layers are generally deposited in a horizontal position. If layers are tilted or folded, it indicates they were deformed after deposition.1
Principle of Lateral Continuity
- Sedimentary beds originate as continuous layers that extend in all directions until they thin out at the edges of the depositional basin or grade into a different sediment type.1
Principle of Cross-Cutting Relationships
- Geologic features that cut across rocks (e.g. faults, igneous intrusions) must form after the rocks they cut through.1
Principle of Inclusions
- The rock mass containing inclusions (e.g. xenoliths) is younger than the inclusions themselves, which must have been incorporated from an older rock mass.1
Unconformities
- An unconformity represents a gap or missing interval in the rock record where deposition was interrupted by erosion or non-deposition.1
- Three types:
- Angular unconformity - Tilted older rocks overlain by younger flat-lying rocks
- Disconformity - Parallel layers with a gap representing a period of erosion
- Nonconformity - Sedimentary rocks over metamorphic/igneous rocks
Applying Relative Dating
- The principles allow geologists to determine the relative age sequence of rock units and geologic events represented, even without numerical dates.
- This establishes a basic geologic timeline for an area.
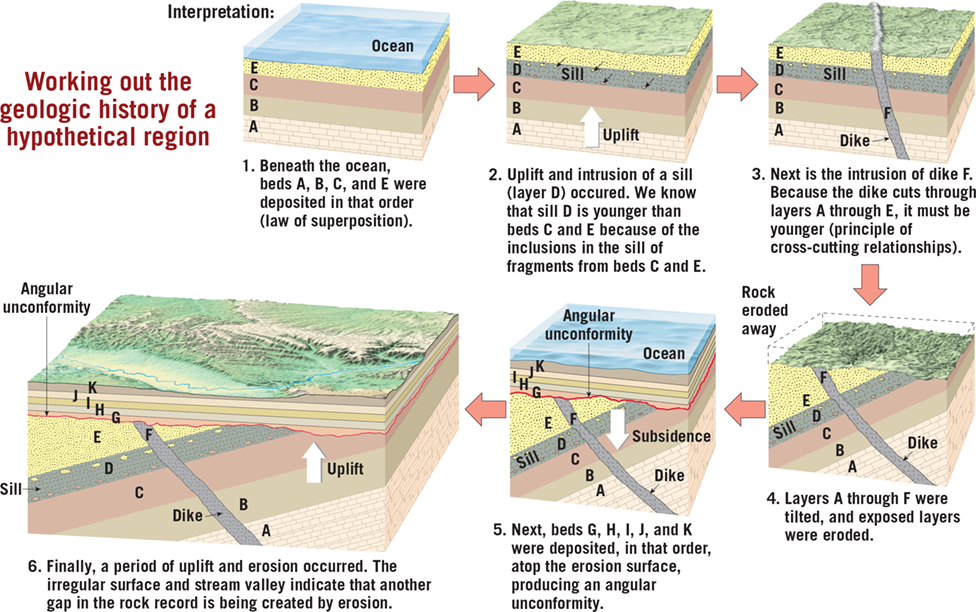
Review 9.1: p.271
1. Distinguish between numerical and relative dates.
- Answer: Numerical dates are specific calendar dates, while relative dates are based on the sequence of events without specific calendar dates.
2. Sketch and label four simple diagrams that illustrate each of the following: superposition, original horizontality, lateral continuity, and cross-cutting relationships.
- Answer:
- Superposition: Layers of rock are deposited on top of each other, with the oldest at the bottom and the youngest at the top.
- Answer:
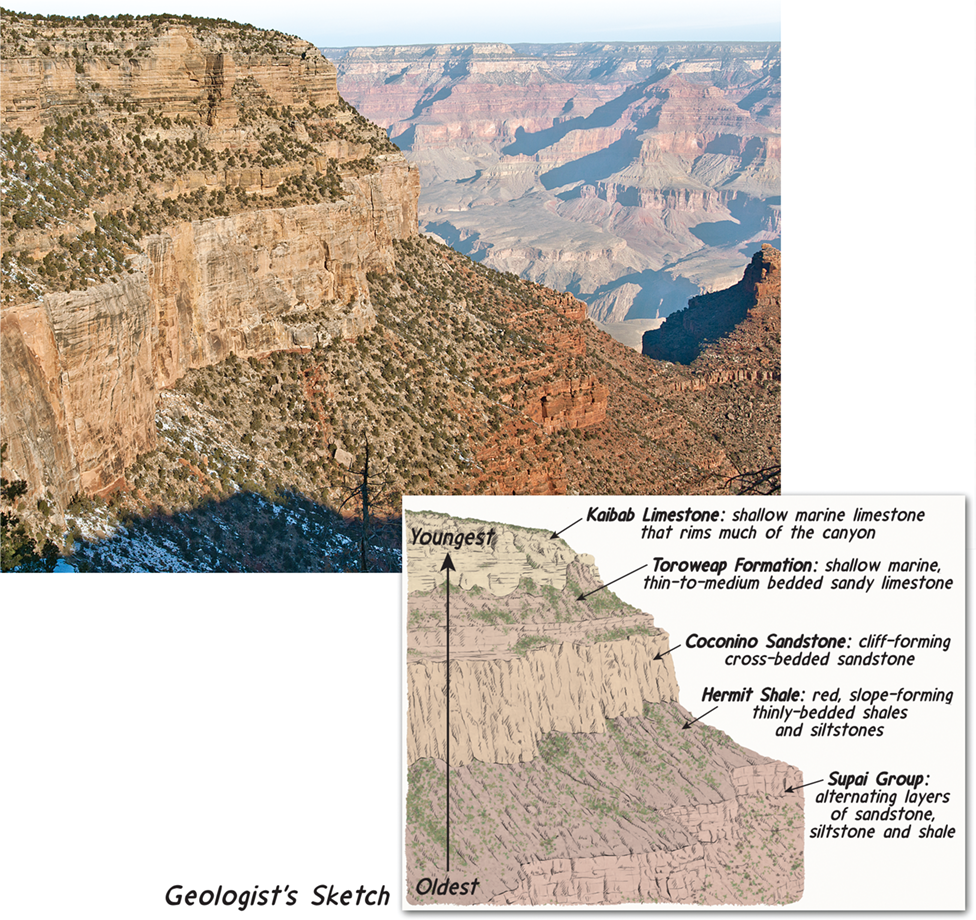
- Original horizontality: Sedimentary layers are initially deposited horizontally.
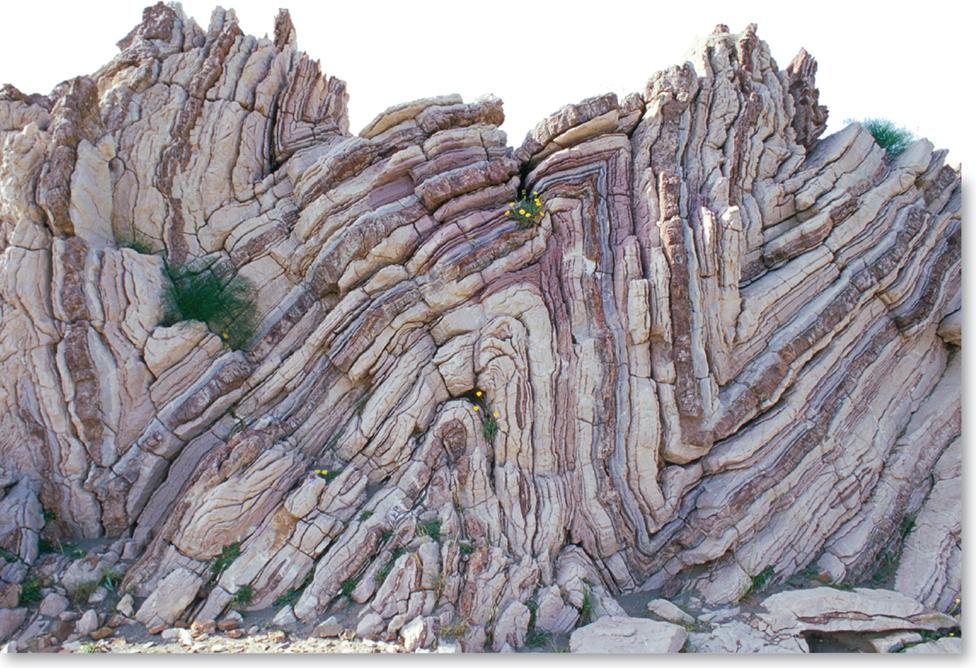
- Lateral continuity: Layers of sedimentary rock extend laterally in all directions until they thin out or grade into a different sediment type.
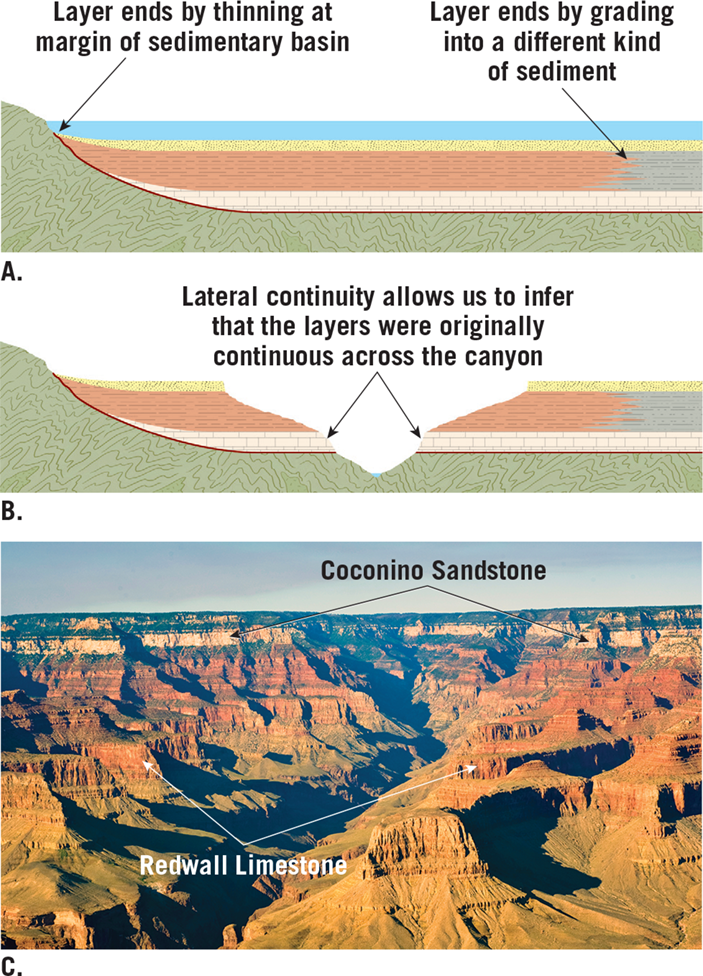
- Cross-cutting relationships: Any feature that cuts across or disrupts another rock layer or feature is younger than the one it cuts across.
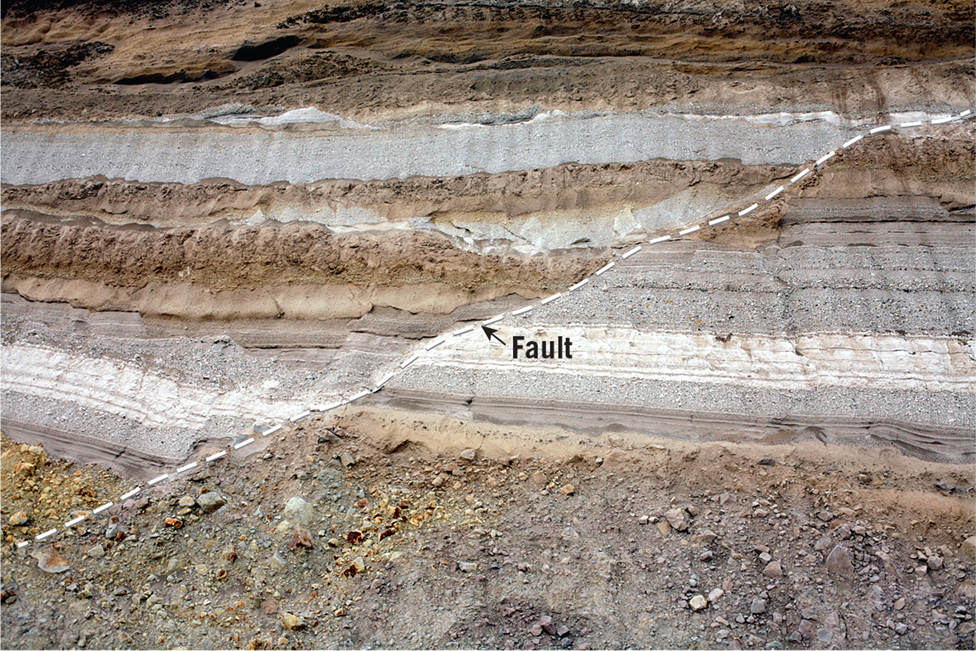
3. What is the significance of an unconformity?
- Answer: An unconformity represents a gap in the geologic record, indicating a period of erosion or non-deposition.
4. Sketch and explain the difference between an angular unconformity and a nonconformity.
- Answer:
- Angular unconformity: Layers of rock are tilted or folded, and then eroded before new sedimentary layers are deposited horizontally on top.
- Answer:
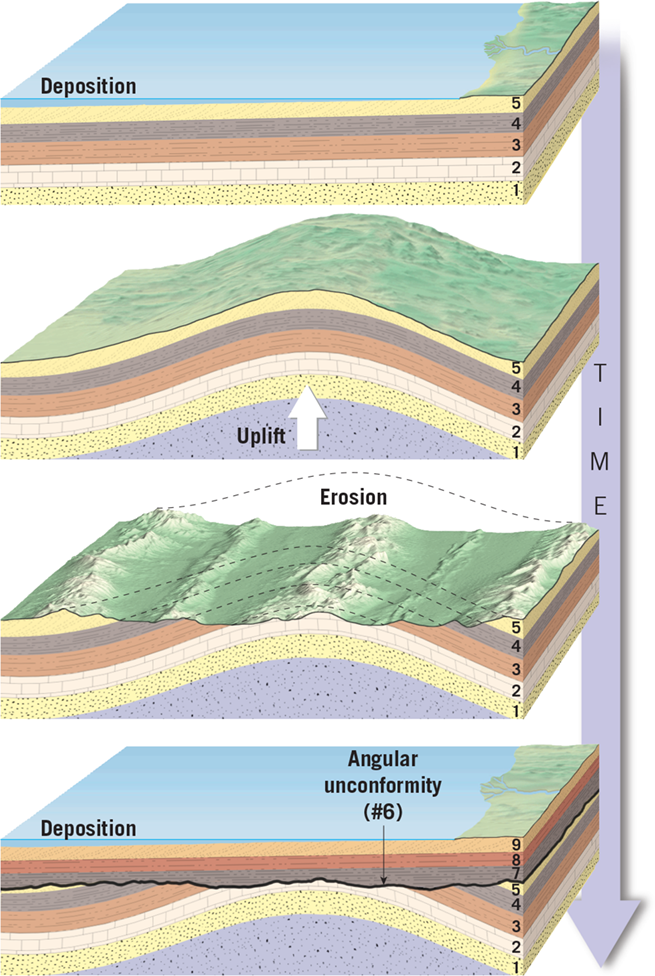
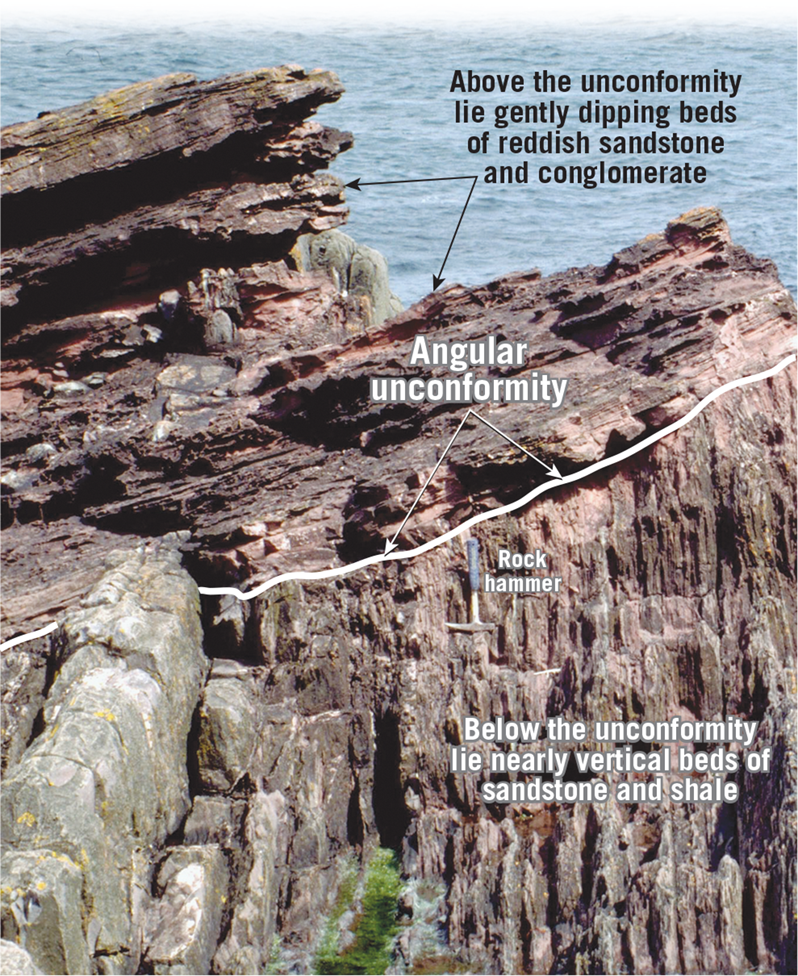
- Nonconformity: Sedimentary rocks overlie igneous or metamorphic rocks, indicating that the sedimentary layers were deposited on top of pre-existing, eroded rocks of a different type.
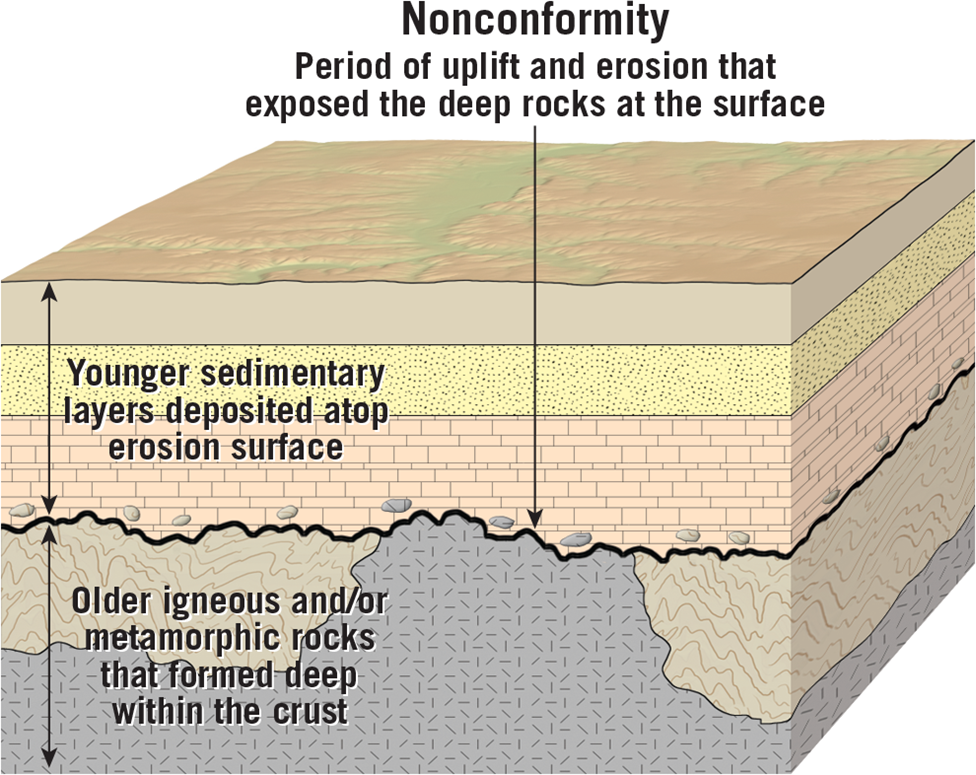
9.2 p.272 Fossils Evidence of Past Life
What is a Fossil?
A fossil is the preserved remains or traces of prehistoric life. Fossils form under specific conditions that allow the remains of organisms to become preserved in sedimentary rock layers.
Conditions Favoring Fossil Preservation
For an organism to become fossilized, two main conditions are required:
- Rapid burial in sediment after death - This protects the remains from destructive surface processes.
- Possession of hard parts (bones, shells, teeth, woody stems) - Soft tissues decay quickly before they can be preserved.
Less than 10% of all organisms that ever existed are represented in the fossil record due to the specific conditions required for fossilization.
Types of Fossils
- Body Fossils
- Preserved actual remains like bones, teeth, shells
- Permineralization - porous tissues filled by mineral deposits
- Petrified wood - permineralized wood turned to stone
- Molds and casts - hollow molds or mineral-filled casts of organisms
- Trace Fossils
- Indirect evidence of life like footprints, burrows, coprolites (fossil dung)
- Carbonization and Impressions
- Carbon films or impressions left in fine sediment by delicate organisms
- Amber
- Organisms trapped and preserved in ancient tree resin
The fossil record is biased towards organisms with hard parts that lived in areas conducive to sedimentation and burial. Soft-bodied organisms are rarely preserved as fossils.13
Fossils provide evidence of the progression and evolution of life over millions of years by showing changes in complexity, diversity, and the appearance and extinction of different life forms through geologic time.13 The fossil record is crucial for understanding prehistoric environments and life on Earth.
9.2 Review
There are several ways an organism can be preserved as a fossil:
- Permineralization - This occurs when mineral-rich groundwater permeates the porous tissues like bone or wood, and minerals precipitate out filling the empty spaces. This turns the organism into a rock-like fossil, such as petrified wood.
- Molds and casts - If a shell or body imprint is left in sediment, it creates a mold. If this hollow mold later gets filled with minerals, it creates a cast fossil which is a replica of the original organism.
- Carbonization - This preserves delicate organisms like leaves or feathers by the remains being encased in fine sediment, squeezed by pressure, leaving just a thin carbon film outline.
- Amber - Some organisms like insects can get trapped and preserved in ancient tree resin that hardens into amber, protecting them from decay.13
Question: List two examples of trace fossils.
Two examples of trace fossils are:
- Tracks/Footprints - Impressions left by an animal's footprints in soft sediment that later hardens into rock.
- Burrows - Tunnels or holes dug into sediment or wood by an animal, that later get filled in by mineral deposits.13
Question: What conditions favor the preservation of an organism as a fossil?
The key conditions that favor an organism being preserved as a fossil are:
- Rapid burial in sediment after death - This protects the remains from destructive surface processes and scavengers.
- Possession of hard parts like shells, bones, teeth - Soft tissues decay too quickly to fossilize easily.
- Anaerobic (oxygen-poor) conditions - Limits bacterial decay and decomposition of the remains.
- Cold, dry conditions - Such as Arctic permafrost, which can preserve remains like woolly mammoths for thousands of years.1245
So in summary, the ideal conditions are rapid burial in an anaerobic, mineral-rich sediment that allows permineralization, in a cold, dry environment that limits decomposition - especially for organisms with hard body parts.
9.3 p.274-275
Correlating Rock Layers Using Fossils
Principle of Fossil Succession
- Fossil organisms succeed each other in a definite and determinable order through geologic time
- Any time period can be recognized by its characteristic fossil content
- This succession of life forms is found in the same order on every continent
Index Fossils
- Widespread geographically but limited to a short span of geologic time
- Presence of an index fossil allows matching of rock layers of the same age
Fossil Assemblages
- If no index fossil is present, a group of fossils (assemblage) can be used to date a rock layer
- Overlapping ranges of multiple fossil species allows more precise dating
Using Fossils as Environmental Indicators
Interpreting Depositional Environments
- Fossil evidence helps deduce the ancient environment the sediments were deposited in
- E.g. Clam fossils in limestone = shallow marine environment
Identifying Shoreline Position
- Thick-shelled fossils = high energy shoreline environment
- Thin, delicate shells = deeper, calmer offshore waters
Determining Water Temperature
- Fossil coral = ancient warm, shallow tropical seas
- Allows interpretation of past climate and water temperatures
In summary, fossils are crucial for correlating rock layers between locations and reconstructing the environmental conditions, climate, and geography that existed when those rocks formed millions of years ago.
9.3 Review p.275
Question: What is the goal of correlation?
The goal of correlation is to match up rock layers of the same age across different geographic areas, even when they are composed of different lithologies (rock types). By correlating rocks from separate locations, geologists can piece together a more comprehensive picture and understanding of Earth's geologic history over a wider region.14
Question: State the principle of fossil succession in your own words.
The principle of fossil succession states that fossil organisms are preserved in sedimentary rock layers in a specific and consistent order that represents their relative timing and evolution through geologic time. Older, more primitive fossil forms will be found in lower rock layers, while younger and more modern fossil forms will be found in higher overlying layers. This ordered succession of fossil types is found globally.135
Question: Along with being important in correlation, how else are fossils useful to geologists?
In addition to their crucial role in correlating rock layers, fossils are also useful to geologists as:
- Environmental indicators - Fossils provide evidence about the ancient environments the sediments were deposited in, such as marine vs terrestrial, shallow vs deep water, warm vs cold climates, etc.13
- Dating rocks - The presence of certain index fossils with short temporal ranges can be used to relatively date when that rock layer was formed.34
- Interpreting evolution - The succession of fossil forms from older to newer shows the pattern and progression of evolution over time.5
- Reconstructing geography - Fossils indicate positions of ancient shorelines, depths of water bodies, locations of landmasses, etc. when combined with other sedimentary evidence.1
So in summary, while critical for correlation, fossils are also invaluable tools that allow geologists to decipher ancient environments, date rocks, trace evolution, and reconstruct the paleogeography of our planet through deep time.
9.4 Numerical Dating with Nuclear Decay
Three Types of Radioactive Decay
- Alpha Decay:
- Definition: Emission of alpha particles (2 protons and 2 neutrons) from the nucleus.
- Effect: Mass number decreases by 4, atomic number decreases by 2.
- Beta Decay:
- Definition: Emission of an electron from the nucleus, converting a neutron to a proton.
- Effect: Mass number remains unchanged, atomic number increases by 1.
- Electron Capture:
- Definition: Capture of an electron by the nucleus, converting a proton to a neutron.
- Effect: Mass number remains unchanged, atomic number decreases by 1.
Use of Radioactive Isotopes in Numerical Dating
- Unstable isotopes (parents) decay into stable isotopes (daughters) at a steady rate.
- By measuring the ratios of parent to daughter isotopes in a sample, the age of the sample can be calculated.
Radiometric Dating
- Half-Life: Time required for half of the nuclei in an unstable isotope to decay.
- Calculation: Age of a sample = (Number of half-lives elapsed) × (Half-life of the isotope).
Unstable Isotopes Used for Radiometric Dating
- Useful isotopes include Rubidium-87, Thorium-232, Uranium-235, Uranium-238, and Potassium-40.
- Potassium-40 is versatile and used for dating rocks younger than 100,000 years.
Sources of Error in Radiometric Dating
- Leakage of parent or daughter isotopes.
- Thermal resetting, especially in the case of Argon-40.
- Weathering or leaching, causing loss of daughter atoms.
Earth's Oldest Rocks
- Rocks exceeding 3.5 billion years in age found globally.
- Radiometric dating confirms immense geologic time.
Dating with Carbon-14
- Radiocarbon Dating: Uses carbon-14 to date organic materials.
- Effective for dating events from the historic past up to 70,000 years ago.
- Carbon-14 is continuously produced in the atmosphere and incorporated into living organisms.
Importance of Carbon-14 Dating
- Valuable for anthropologists, archaeologists, historians, and geologists studying recent Earth history.
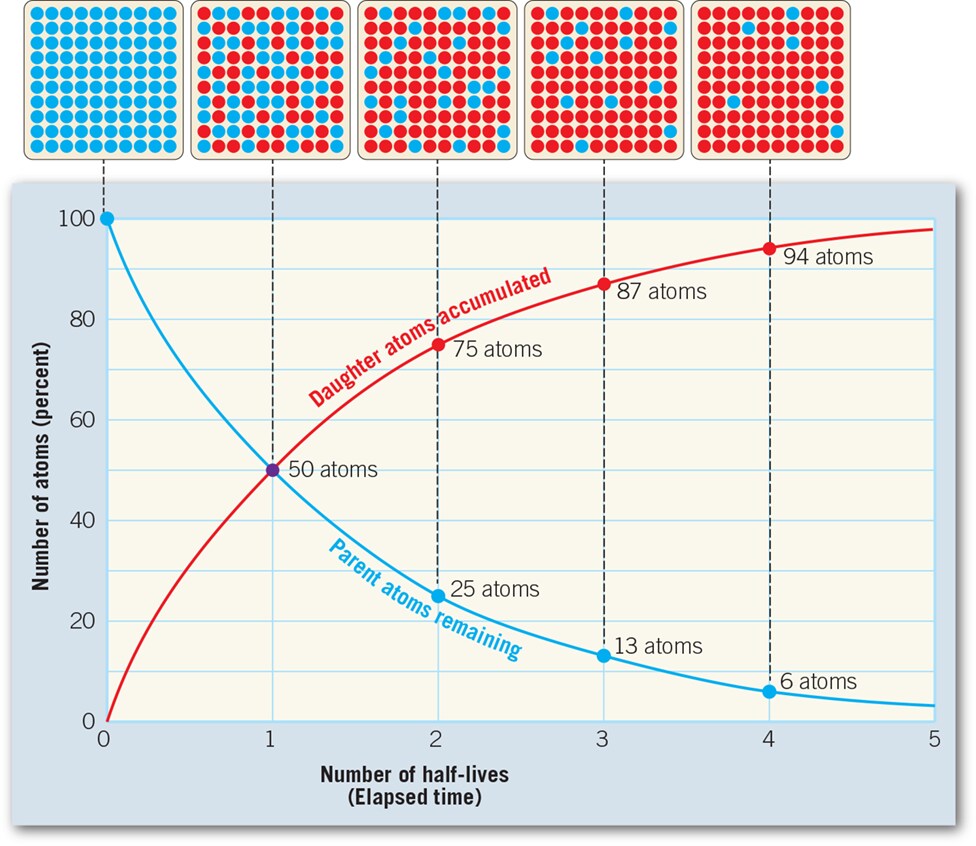
Review 9.4
- List three types of radioactive decay. For each type, describe how the atomic number and atomic mass change.
- Alpha Decay:
- Change: Atomic mass decreases by 4, atomic number decreases by 2.
- Beta Decay:
- Change: Atomic mass remains unchanged, atomic number increases by 1.
- Electron Capture:
- Change: Atomic mass remains unchanged, atomic number decreases by 1.
- Alpha Decay:
- Sketch a simple diagram to explain the idea of half-life.
- [Sketch of a graph with time on the x-axis and remaining radioactive material on the y-axis. The graph shows a steady decrease in the amount of radioactive material over time, with each half-life representing a halving of the remaining material.]
- For what time span does radiocarbon dating apply?
- Answer: Radiocarbon dating can be used to date events from the historic past as well as those from very recent geologic history, typically up to around 70,000 years ago.
9.5 Determining Numerical Dates for Sedimentary Strata
Reliability of Numerical Dates for Sedimentary Rocks
- Explanation: Determining reliable numerical dates for layers of sedimentary rock poses challenges due to the inability to directly date sedimentary rocks using radiometric methods.
Radiometric Dating Limitations for Sedimentary Rocks
- Explanation: Sedimentary rocks rarely yield accurate radiometric ages because their grains are not the same age as the rock itself, having been weathered and eroded from rocks of diverse ages. Similarly, radiometric dates from metamorphic rocks may not represent the rock's initial formation but rather subsequent metamorphic phases.
Relationship to Igneous Rocks
- Explanation: Numerical dates for sedimentary layers are often determined by their relationship to datable igneous rocks. Geologists relate sedimentary strata to nearby igneous masses to establish numerical dates.
Example: Dating Sedimentary Strata
- Explanation: Radiometric dating of volcanic ash beds and intruding dikes provides numerical dates for sedimentary layers. Layers above the ash are younger, while those below are older. Dikes are younger than the rocks they cut through but older than the rocks they intrude.
Combining Methods for Dating
- Explanation: Geologists combine laboratory dating methods with relative dating principles to estimate the ages of sedimentary strata. This involves examining relationships between sedimentary layers and datable igneous rocks to bracket specific time periods in Earth's history.
Conclusion:
Determining numerical dates for sedimentary strata involves overcoming the limitations of radiometric dating by relying on relationships with igneous rocks. By combining laboratory dating techniques with field observations, geologists can estimate the ages of sedimentary layers and gain insights into Earth's history.
9.5 Review p. 282
- Briefly explain why it is often difficult to assign a reliable numerical date to a sample of sedimentary rock.
- Answer: Assigning a reliable numerical date to sedimentary rock samples is challenging because sedimentary rocks rarely contain minerals that can be directly dated using radiometric methods. The grains within sedimentary rocks are typically not the same age as the rock itself, having been weathered and eroded from various sources, making direct dating unreliable.
- How might a numerical date for a layer of sedimentary rock be determined?
- Answer: Numerical dates for sedimentary rock layers are often determined by examining their relationship to nearby igneous rocks. Geologists utilize radiometric dating techniques on igneous rocks that intrude or overlie sedimentary layers. By dating volcanic ash beds or igneous dikes within or adjacent to sedimentary layers, geologists can establish numerical ages for those sedimentary strata based on their relative positions to the dated igneous rocks.
9.6 The Geologic Time Scale
Structure of the Geologic Time Scale
- Explanation: The geologic time scale divides Earth's 4.6-billion-year history into various units, with eons representing the broadest expanses of time, followed by eras, periods, and epochs.
Basic Time Units:
- Eons:
- Definition: The broadest divisions of time, with the Phanerozoic eon (541 million years ago to present) containing abundant fossil evidence.
- Eras:
- Definition: Subdivisions of eons, including the Paleozoic, Mesozoic, and Cenozoic eras, characterized by significant changes in life-forms.
- Periods:
- Definition: Subdivisions of eras, such as the Paleozoic with seven periods, representing more specific changes in life evolution.
- Epochs:
- Definition: Smaller units within periods, providing finer detail of geological time, particularly in the Cenozoic era.
Precambrian Time
- Explanation: Represents the vast expanse of Earth's history before the Cambrian period, comprising the Archean and Proterozoic eons.
- Challenges: Difficult to subdivide due to lack of detailed knowledge and the absence of abundant fossil evidence.
Dynamic Nature of the Time Scale
- Explanation: The geologic time scale is continually refined as new evidence and understanding of Earth's history emerge.
- Updates: The International Commission on Stratigraphy periodically updates the time scale to include changes in unit names and boundary age estimates.
Informal Terms
- Explanation: Terms like "Precambrian" and "Hadean" are informally used despite not being officially recognized on the geologic time scale.
- Purpose: Aid effective communication in the geosciences despite lacking formal status.
Anthropocene
- Explanation: Some scientists propose the Anthropocene as a new epoch, reflecting human-caused global environmental changes.
- Debate: There's ongoing debate about when the Anthropocene began and whether it should be officially recognized as a geologic epoch.
- Dynamic Tool: Recognizes the evolving nature of Earth's history and our understanding of it.
Conclusion:
The geologic time scale provides a framework for understanding Earth's complex history, with divisions ranging from eons to epochs. It evolves as our knowledge advances, reflecting the dynamic nature of Earth's geological processes and the impact of human activities.
9.6 Review p.
- List the four basic units that make up the geologic time scale.
- Answer: The four basic units of the geologic time scale are eons, eras, periods, and epochs.
- What term applies to all of geologic time prior to the Phanerozoic eon? Why is this span not divided into epochs as is the Phanerozoic eon?
- Answer: The term "Precambrian" applies to all of geologic time prior to the Phanerozoic eon. This span is not divided into epochs like the Phanerozoic eon because the Precambrian lacks detailed knowledge and abundant fossil evidence. The rocks from this time are highly distorted and metamorphosed, making interpretation difficult.
- Explain why scientists occasionally change the geologic time scale.
- Answer: Scientists occasionally change the geologic time scale to incorporate new discoveries, refine dating techniques, and update unit names and boundary age estimates. This ensures that the time scale remains accurate and reflects the latest understanding of Earth's history.
Question:
- The accompanying diagram is a cross section of a hypothetical area. Place the lettered features in the proper sequence, from oldest to youngest. Where in the sequence can you identify an unconformity? Which principles did you use to establish the sequence?
Question
- Measurements of zircon crystals containing trace amounts of uranium from a specimen of granite yield parent/daughter ratios of 25 percent parent (uranium-235) and 75 percent daughter (lead-206). The half-life of uranium-235 is 704 million years. How old is the granite?
Question
Express the numerical age of the sandstone layer in the diagram as accurately as possible.
Chapter 16
The Hydrologic Cycle
Earth's Water Reservoirs
- The vast majority (96.5%) of Earth's water is stored in the global oceans145.
- The remaining freshwater is found in glaciers and ice sheets (1.7%), groundwater (1.7%), lakes and rivers (0.01%), and the atmosphere (0.001%)145.
Paths of Water in the Hydrologic Cycle
- The hydrologic cycle is powered by energy from the Sun and involves the continuous circulation of water through Earth's land, oceans, and atmosphere12345.
- Water enters the atmosphere primarily through evaporation from the oceans, with a smaller contribution from transpiration by plants1245.
- Winds transport water vapor, which condenses to form clouds and eventually precipitates back to the Earth's surface12345.
- Precipitation that falls on land can either infiltrate the soil and become groundwater, run off into streams and rivers, or evaporate back into the atmosphere12345.
- Groundwater can slowly seep into lakes, streams, and the ocean, completing the cycle12345.
Water Balance
- The total amount of water vapor in the atmosphere remains relatively constant, so the average annual precipitation worldwide must equal the amount of water evaporated145.
- However, precipitation exceeds evaporation over land, while evaporation exceeds precipitation over the oceans145.
- This imbalance is resolved by the vast amount of water (36,000 cubic km annually) that flows from land back to the ocean145.
Importance of the Hydrologic Cycle
- The hydrologic cycle is a crucial process that shapes Earth's land surface through erosion, flooding, and groundwater formation145.
- It also provides the water resources necessary to sustain life and human activities on land1245.
- Evaporation: Liquid water changes into water vapor and enters the atmosphere from oceans and land.
- Transpiration: Plants release water vapor into the atmosphere.
- Precipitation: Water vapor condenses and falls back to Earth's surface as rain, snow, sleet, or hail.
- Infiltration: Precipitation that falls on land may soak into the ground, moving laterally and eventually seeping into lakes, streams, or the ocean.
- Runoff: Excess water flows over the surface into lakes and streams when infiltration capacity is exceeded.
- Storage in Glaciers: Precipitation in cold regions accumulates as snow or ice, forming glaciers that store large quantities of water on land.
- Evapotranspiration: Combined process of evaporation from soil, lakes, and streams, along with transpiration from plants, transferring water back to the atmosphere.
16.1 Review
- Describe or sketch the movement of water through the hydrologic cycle. Once precipitation has fallen on land, what paths might the water take?
- In the hydrologic cycle, water moves through various stages:
- Evaporation: Liquid water from oceans and land surfaces turns into water vapor and rises into the atmosphere.
- Transpiration: Plants release water vapor through their leaves.
- Condensation: Water vapor condenses to form clouds.
- Precipitation: Condensed water falls back to Earth's surface as rain, snow, sleet, or hail.
- Infiltration: Precipitation that lands on the ground may seep into the soil.
- Runoff: Excess water flows over the surface into rivers, lakes, and oceans.
- Storage in Glaciers: Some precipitation accumulates as snow and forms glaciers, storing water on land.
- Evapotranspiration: Combined process of evaporation from soil, lakes, and streams, along with transpiration from plants, returns water vapor to the atmosphere.
- In the hydrologic cycle, water moves through various stages:
- Once precipitation has fallen on land, water might follow various paths:
- Soak into the ground (infiltration)
- Flow over the surface (runoff)
- Be absorbed by plants and later released back into the atmosphere (transpiration)
- Accumulate in rivers, lakes, or groundwater reservoirs
- Eventually return to the ocean through runoff or groundwater flow.
- What is meant by the term evapotranspiration?
- Evapotranspiration refers to the combined process of evaporation and transpiration. It involves the transfer of water from the Earth's surface, such as soil, lakes, and streams, into the atmosphere. Evaporation occurs when liquid water turns into water vapor from surfaces like soil and bodies of water. Transpiration occurs when plants absorb water through their roots and release water vapor through their leaves into the atmosphere. Together, evaporation and transpiration contribute to the water cycle by returning water vapor to the atmosphere.
- Over the oceans, evaporation exceeds precipitation, yet sea level does not drop. Explain why.
- Although evaporation exceeds precipitation over the oceans, sea level does not drop because water is constantly cycled back to the oceans through other pathways. The excess water evaporated from the oceans is replenished by precipitation falling on land. This water eventually makes its way back to the oceans through runoff, groundwater flow, and rivers. Additionally, melting ice from glaciers and polar ice caps also contributes to maintaining sea levels. Overall, the hydrologic cycle ensures that water is continuously redistributed, preventing a significant drop in sea level despite regional differences in evaporation and precipitation.
The hydrologic cycle -- The Earth's water transfer system is called the hydrologic cycle. The water system is powered by the sun. 97.2% of the Earth's total water is in the oceans. 2.2% is in ice caps and glaciers. 0.6% is in groundwater, rivers, lakes and the atmosphere.
Freshwater budget -- Groundwater is 14% of freshwater in hydrosphere. The average rate of groundwater exchange is 280 years. Exchange means the transfer from the atmosphere to the ground and back to the atmosphere. This exchange rate gives an indication that groundwater movement is slow.
Water table -- The water table defines the top of the zone of saturation, where groundwater occurs. Porosity governs the amount of groundwater that a rock material can hold. The water table is not flat. The water table is higher under hills than under valleys. Groundwater flow is impeded by indirect flow around grains.
Permeability -- Permeability defines the ability of a porous rock to transmit a fluid. Permeable layers are called aquifers. Impermeable layers are called aquicludes. Springs arise from a perched water table. Otherwise groundwater discharges into streams as a consequence of flowing from a higher water under hills towards the lower water table in valleys. If water discharges into a stream, the water table comes to surface right at the stream. In this case the water table slopes downhill toward the stream.
Mining Groundwater -- Wells are drilled into the zone of saturation below the water table. When wells are pumped, the water flows to the well which locally depresses the water table (drawdown) and creates a cone of depression.
Groundwater is an exhaustible resource.
River Systems (Section 16.2)
1. Nature of Drainage Basins and River Systems:
- Drainage Basin Definition: An area of land drained by a river and its tributaries.
- River System Definition: Includes the network of stream channels and the entire drainage basin.
- Key Concepts:
- Drainage basin bounded by a divide, with an outlet at a lower elevation.
- Drainage divides can vary in scale from small ridges to continental divides.
- Example: The Mississippi River drainage basin is the largest in North America.
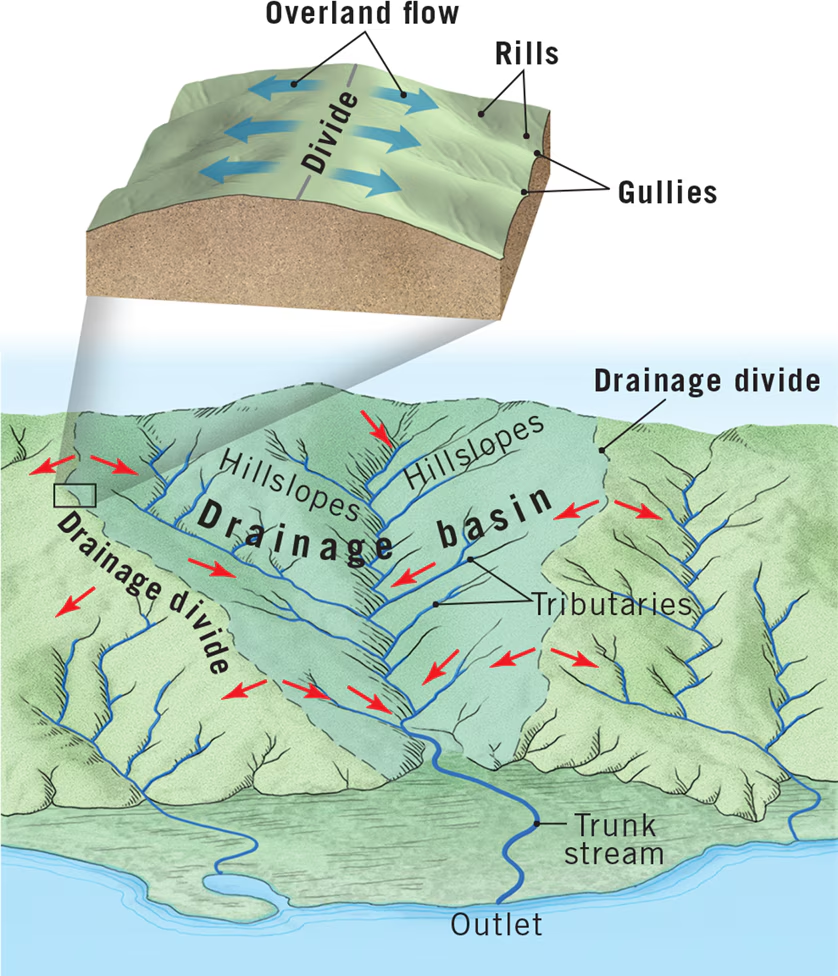
2. Factors Influencing Runoff:
- Factors:
- Intensity and duration of rainfall.
- Soil moisture content.
- Surface material permeability.
- Slope of the land.
- Vegetation type and extent.
- Runoff Dominance: Occurs when surface material is impermeable or saturated, leading to high runoff.
3. Processes of Stream Formation:
- Sheet Flow: Initial runoff across slopes.
- Rills: Tiny channels formed from sheet flow.
- Gullies: Channels formed by merging rills.
- Streams and Rivers: Formed by merging gullies and tributaries.
4. Sources of Streamflow:
- Humid Regions: Streamflow from overland flow and groundwater.
- Arid Regions: Stream loses water to groundwater due to lower water table level.
5. Drainage Patterns:
- Dendritic Pattern: Irregular tributary streams resembling tree branches. Most common. Because the surface material is essentially uniform in its resistance to erosion, it does not control the pattern of streamflow. Rather, the pattern is determined chiefly by the direction of slope of the land.
- Radial Pattern: Streams diverge from a central area like spokes on a bike tire, common in volcanic regions.
- Rectangular Pattern: Streams with right-angle bends due to underlying structural features.
- Trellis Pattern: Parallel tributary streams, common in areas with alternating bands of resistant rock.
6. River Zones and Sediment Dynamics:
- Zones: Sediment production, transport, and deposition.
- Sediment Production: Occurs in headwaters through weathering and erosion.
- Sediment Transport: Sediment moves through trunk streams.
- Sediment Deposition: Occurs at river mouths, forming deltas or coastal features.
7. River Evolution:
- Headward Erosion: Lengthening of streams by erosion of their heads upslope.
- Antecedent Streams: Streams that maintain their course despite uplift.
- Superposed Streams: Streams that erode through existing structures due to sedimentary layering.
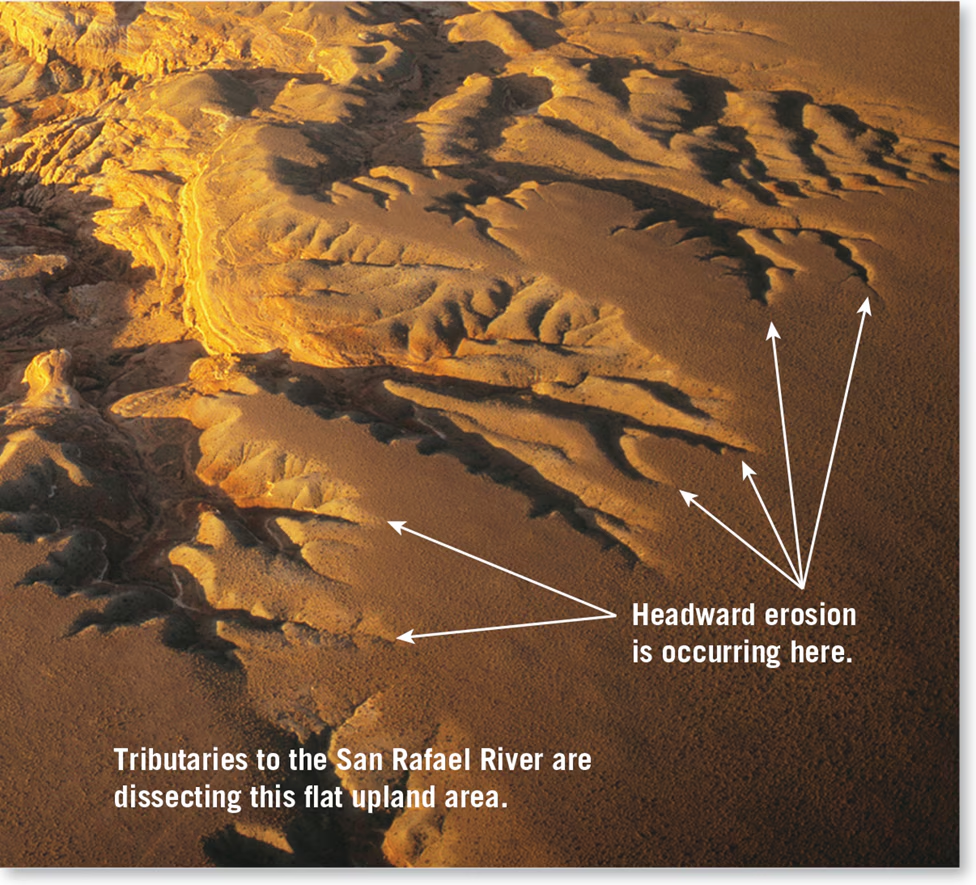
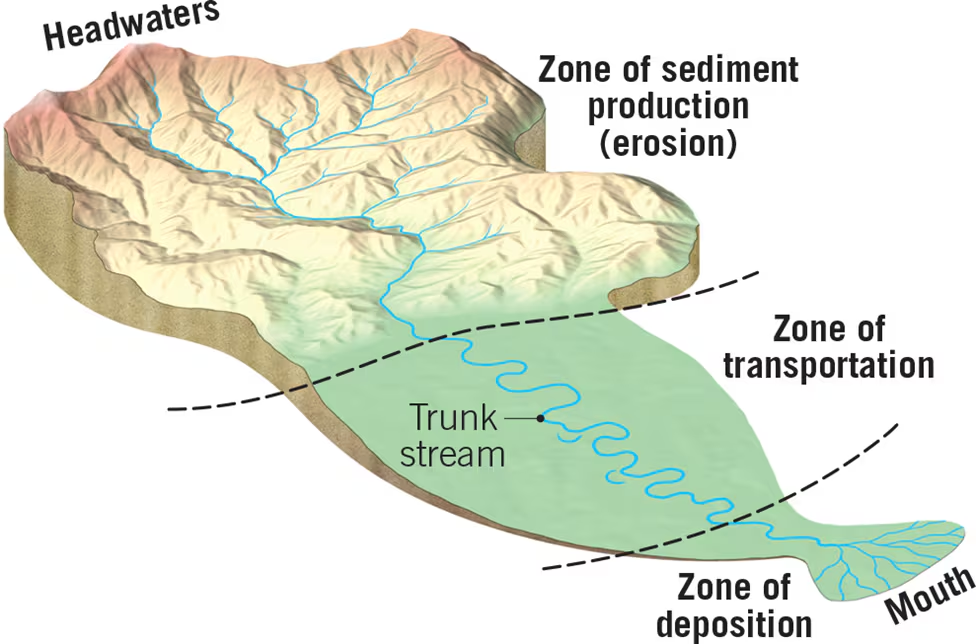
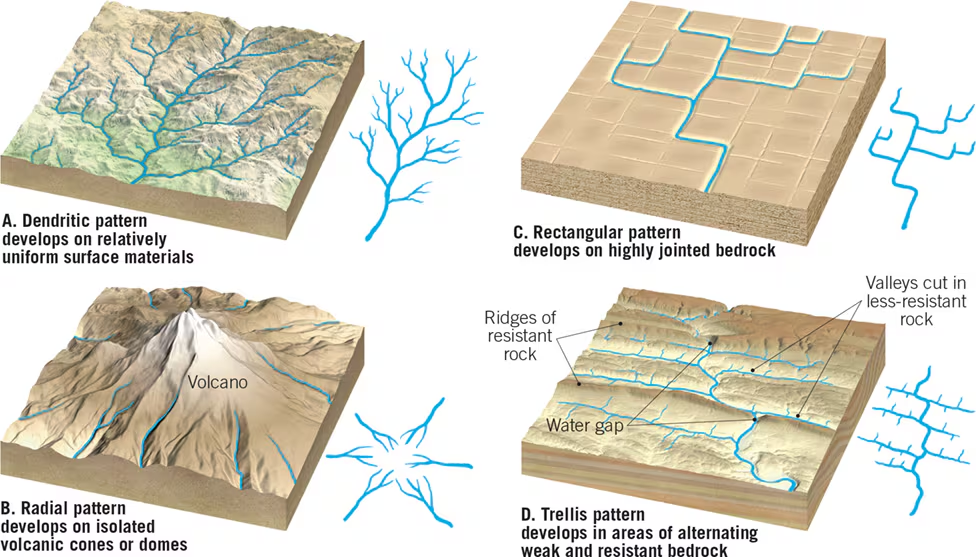
Summary: 16.2
Understanding river systems involves grasping concepts such as drainage basins, runoff factors, stream formation processes, sources of streamflow, drainage patterns, sediment dynamics, and river evolution. Each aspect contributes to the complex behavior and morphology of rivers, shaping landscapes over time.
16.2 Review Questions
Factors influencing infiltration and runoff variability:
- Rainfall Intensity and Duration: Heavy rainfall over a short period increases runoff, while light rainfall over a longer duration promotes infiltration.
- Soil Type and Moisture Content: Sandy soils facilitate infiltration, while clayey soils can lead to surface runoff.
- Topography: Steeper slopes enhance runoff due to gravity, while flat areas allow more time for infiltration.
- Vegetation Cover: Dense vegetation slows down runoff by intercepting rainfall and promoting infiltration through root systems.
- Human Activities: Urbanization, deforestation, and land use changes can increase surface runoff by creating impermeable surfaces.
Simple sketch of a drainage basin and a divide:
- Drainage Basin: Area drained by a river and its tributaries.
- Divide: Imaginary line separating two adjacent drainage basins.
Three main parts (zones) of a river system:
- Zone of Sediment Production: Located in the headwaters region where erosion dominates, contributing sediment to the river.
- Zone of Sediment Transport: The main channel network where sediment is transported downstream by the river.
- Zone of Sediment Deposition: Where the river reaches a body of water, slowing down and depositing sediment, forming deltas or coastal features.
Brief description of the four main stream patterns and their causes:
- Dendritic Pattern: Irregular tributary streams resembling tree branches. Occurs in areas with uniform underlying geology and slope.
- Radial Pattern: Streams diverge from a central area like spokes from a wheel hub. Common in volcanic regions.
- Rectangular Pattern: Streams with right-angle bends due to underlying structural features like joints or faults.
- Trellis Pattern: Parallel tributary streams resembling a garden trellis. Forms in areas with alternating bands of resistant and less-resistant rock, often seen in folded mountain ranges.
16.3 Streamflow Characteristics
1. Streamflow and its Characteristics:
- Definition: Streamflow refers to the movement of water within river channels under the influence of gravity. It encompasses the various behaviors and properties of water as it moves through channels, including velocity, turbulence, and erosion capacity.
- Flow Types:
- Laminar Flow: This type of flow occurs when water moves smoothly in parallel layers, with minimal mixing between adjacent layers. It is characterized by streamlined paths and low turbulence. Laminar flow is typically observed in very slow-moving streams and is governed by viscosity and inertia.
- Turbulent Flow: Turbulent flow is characterized by irregular and chaotic movement of water, with mixing occurring between adjacent layers. It is commonly observed in natural streams and rivers, especially in areas with high velocities or obstacles. Turbulence contributes to increased erosion and sediment transport capacity.
2. Factors Influencing Streamflow:
- Flow Velocity: Flow velocity refers to the speed at which water moves within a stream channel. It is influenced by various factors including channel gradient, shape, size, roughness, and discharge. Higher velocities are associated with increased turbulence and erosion potential.
- Gradient: Gradient, also known as slope, represents the change in elevation of a stream channel over a given distance. Steeper gradients result in higher flow velocities and increased erosional power, while gentler gradients lead to slower velocities and reduced erosional capacity.
- Channel Shape: The shape of the stream channel cross-section significantly impacts flow characteristics. Channel shape influences the wetted perimeter, which is the portion of the channel in contact with water. A smaller wetted perimeter reduces frictional resistance and promotes higher flow velocities.
- Channel Size and Roughness: Larger channels generally exhibit higher flow velocities compared to smaller channels due to increased cross-sectional area and discharge. Additionally, the roughness of the channel bed and banks affects flow velocity, with smoother surfaces reducing frictional resistance and enhancing flow.
- Discharge: Discharge refers to the volume of water passing a specific point in the stream channel over a given time period. It is calculated by multiplying the cross-sectional area of the channel by the flow velocity. Discharge is a key determinant of a stream's capacity for erosion, sediment transport, and hydraulic efficiency.
3. Monitoring Streamflow:
- The monitoring of streamflow involves the collection and analysis of data related to flow velocity, discharge, and river stage. This data is typically obtained through a network of stream gaging stations maintained by organizations such as the U.S. Geological Survey (USGS).
- Streamflow data is essential for various purposes, including flood forecasting, water resource management, infrastructure design, and environmental monitoring. It provides valuable insights into the behavior and dynamics of river systems, aiding in decision-making and risk assessment.
4. Changes from Upstream to Downstream:
- Longitudinal Profile: The longitudinal profile of a stream represents a cross-sectional view of its channel from its source (headwaters) to its mouth (where it joins another body of water). It typically exhibits a gradual decrease in gradient from upstream to downstream.
- Gradient Decrease: As streams flow downstream, the slope of the channel, or gradient, generally decreases. This reduction in gradient is accompanied by an increase in discharge and channel size. While the decrease in gradient lowers the energy available for erosion, the larger channel dimensions and increased discharge compensate for this reduction, resulting in similar or higher flow velocities downstream.
- Sediment Changes: Along most rivers, changes in sediment characteristics occur from upstream to downstream. Sediment size tends to decrease downstream, leading to smoother channel beds and increased hydraulic efficiency. This downstream fining of sediment particles is a result of selective transport, with coarser particles settling out closer to the source while finer particles remain in suspension and travel further downstream.
Summary:
Understanding streamflow characteristics involves considering factors such as flow velocity, gradient, channel shape, size, roughness, discharge, and sediment dynamics. Monitoring streamflow through gaging stations provides valuable data for various applications, including flood forecasting and water resource management. Changes in stream characteristics from upstream to downstream, including gradient decrease and sediment changes, contribute to the overall behavior and morphology of river systems.
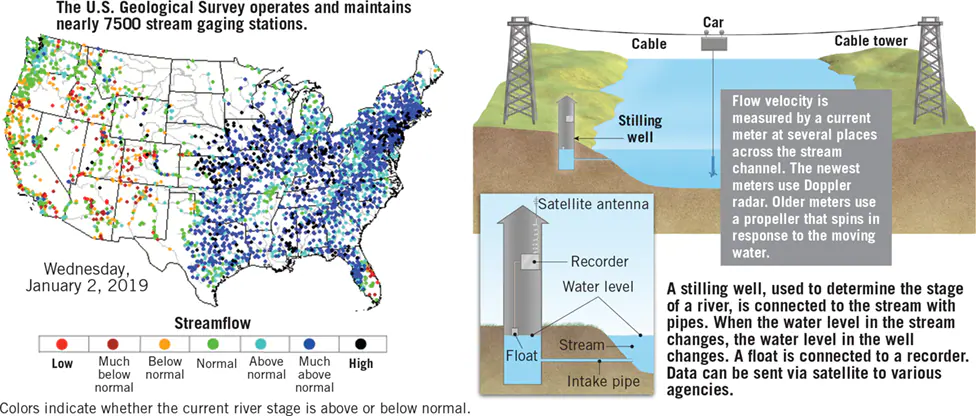
16.3 Review Questions
1. Contrast laminar flow and turbulent flow:
Laminar Flow:
- Description: Laminar flow occurs when water moves smoothly in parallel layers, with minimal mixing between adjacent layers.
- Characteristics:
- Smooth, streamlined paths.
- Low turbulence.
- Predominant in slow-moving streams.
- Factors: Governed by viscosity and inertia.
- Effect: Minimal mixing between layers, resulting in uniform flow.
Turbulent Flow:
- Description: Turbulent flow is characterized by irregular and chaotic movement of water, with mixing occurring between adjacent layers.
- Characteristics:
- Irregular and chaotic movement.
- High turbulence.
- Common in natural streams and rivers.
- Factors: Influenced by obstacles, high velocities, and changes in channel geometry.
- Effect: Enhanced mixing between layers, leading to increased erosion and sediment transport capacity.
2. What is a longitudinal profile:
- A longitudinal profile represents a cross-sectional view of a stream or river channel from its source (headwaters) to its mouth (where it joins another body of water). It depicts changes in elevation along the length of the channel and provides insights into the slope, gradient, and overall morphology of the stream.
3. Changes in channel width, depth, flow velocity, and discharge between headwaters and mouth of a stream:
- Channel Width: Typically increases from headwaters to mouth. This occurs as additional tributaries join the main channel, contributing to increased water volume and channel width.
- Channel Depth: Generally increases downstream due to the accumulation of water from tributaries and increased discharge. Additionally, deeper channels are capable of carrying larger volumes of water.
- Flow Velocity: Initially higher in headwaters due to steeper gradients and narrower channels. However, as the stream progresses downstream, flow velocity may increase due to the larger channel dimensions and increased discharge compensating for reduced gradient.
- Discharge: Increases downstream as additional tributaries contribute water to the main channel. Discharge is calculated as the product of channel cross-sectional area and flow velocity, thus reflecting the combined effects of channel width, depth, and flow velocity.
4. Explanation for changes:
- Gradient Decrease: The reduction in channel gradient downstream leads to decreased energy available for erosion, resulting in a decrease in flow velocity. However, the increase in channel dimensions and discharge downstream compensates for this reduction, maintaining or even increasing flow velocity.
- Sediment Deposition: As flow velocity decreases downstream, the stream's capacity to transport sediment diminishes, leading to sediment deposition. This deposition contributes to the widening and deepening of the channel.
- Tributary Contributions: Additional water inputs from tributaries contribute to increased discharge and channel dimensions downstream. As a result, channel width, depth, and discharge generally increase from headwaters to mouth.
16.4 Running Water
Stream Erosion: Stream erosion refers to the process by which running water removes soil and weathered rock from the Earth's surface, primarily through the action of flowing water.
Raindrop Erosion: Raindrops dislodge sediment particles, initiating erosion, particularly on saturated ground where water cannot infiltrate, leading to sheet flow and the formation of small channels or rills.
Channel Erosion: Confined flow in a channel enhances erosion, influenced by slope, discharge, and the relative resistance of bank and bed material. Unconsolidated materials are more easily eroded than bedrock.
Types of Channel Erosion:
- Quarrying: Removal of blocks from the stream bed, aided by fracturing and weathering, mainly due to impact forces exerted by flowing water.
- Abrasion: Bed and banks of a channel are bombarded by sediment particles carried by the flow, leading to erosion and smoothing of sediment grains.
- Corrosion: Soluble rock, such as limestone, is gradually dissolved by flowing water, a chemical weathering process.
Transport of Sediment:
Definition: The process by which streams carry and transport sediment, which includes dissolved, suspended, and bed loads.
Dissolved Load: Consists of minerals dissolved in water, acquired from groundwater and precipitation, with velocity having minimal effect on transport.
Suspended Load: Mainly comprises fine sand, silt, and clay particles carried in suspension, influenced by flow velocity and settling velocity. Settling velocity determines whether particles remain suspended or settle to the streambed.
Bed Load: Sediment too large to be carried in suspension moves along the streambed through rolling, sliding, or saltation, influenced by channel characteristics and discharge.
Capacity and Competence:
Capacity: Maximum load of solid particles a stream can transport per unit time, influenced by discharge. Large rivers with high flow velocities have large capacities.
Competence: Measure of a stream's ability to transport particles based on size, determined by flow velocity. Higher velocity increases competence exponentially, affecting the transport of larger particles.
Deposition of Sediment:
Definition: Deposition refers to the process by which sediment carried by streams settles out of the flowing water, primarily due to a reduction in flow velocity.
Sorting: Sediment particles of various sizes are separated during transport, with larger particles settling first as flow velocity decreases. This process leads to the formation of depositional features composed of sorted sediment.
Alluvium: General term for sediment deposited by streams, forming various depositional features within stream channels, on the valley floor, and at the mouth of the stream.
16.4 Review
- Describe two processes by which streams cut channels in bedrock.Answer: Streams cut channels in bedrock through quarrying, involving the removal of blocks from the stream bed aided by fracturing and weathering, and abrasion, where the bed and banks are eroded by sediment particles carried by the flow.
- In what three ways does a stream transport its load? Which part of the load moves most slowly?Answer: A stream transports its load through dissolved load (minerals dissolved in water), suspended load (fine particles carried in suspension), and bed load (coarser sediment moving along the streambed). The bed load moves most slowly.
- What is settling velocity? What factors influence settling velocity? Does settling velocity affect the dissolved load?Answer: Settling velocity is the speed at which a particle falls through still water. Factors influencing settling velocity include particle size, shape, and specific gravity. Settling velocity primarily affects the transport of suspended and bed loads, not the dissolved load.
16.5 Stream Channels
1. Bedrock Channels:
- Definition: Channels cut into solid rock, typically forming in headwaters with steep slopes.
- Characteristics:
- Energetic flow transports coarse particles that actively abrade the channel.
- May feature steps (steep segments with rapids) and pools (flatter segments with accumulated alluvium).
- Channel pattern often controlled by underlying geologic structure, resulting in winding or irregular channels.
- Example: Streams flowing over steep terrain in mountainous regions.
2. Alluvial Channels:
- Definition: Channels composed mainly of unconsolidated sediment (alluvium) and undergo significant changes in shape due to erosion, transportation, and deposition of sediments.
- Characteristics:
- Shape influenced by sediment size, channel gradient, and discharge.
- Two common types: meandering channels and braided channels.
- Example: Many lowland rivers and streams with gentle gradients.
3. Meandering Channels:
- Definition: Streams characterized by sweeping bends called meanders, flowing in relatively deep, smooth channels and primarily transporting mud, sand, and fine gravel.
- Characteristics:
- Meanders evolve over time as bends migrate across the floodplain.
- Erosion focused at the outside of meanders (cut bank), while sediment deposition occurs on the inside (point bars).
- Bends migrate laterally and downstream over time, forming oxbow lakes through cutoff processes.
- Example: Lower Mississippi River.
4. Braided Channels:
- Definition: Complex network of converging and diverging channels threading among islands or gravel bars, formed where a large portion of a stream’s sediment load consists of coarse material and the stream has a highly variable discharge.
- Characteristics:
- Channels wide and shallow due to erodible bank material.
- Form in environments with high sediment supply and variable flow rates.
- Sediment deposition forms bars, causing flow to split into multiple paths around them.
- Example: Streams at the terminus of glaciers with large seasonal discharge variations.
Key Points:
- Bedrock channels cut into solid rock, often in headwaters with steep slopes, while alluvial channels are composed of unconsolidated sediment and undergo shape changes.
- Meandering channels feature sweeping bends (meanders) and lateral migration, while braided channels consist of multiple, interwoven paths separated by bars.
- Channel characteristics influenced by factors such as sediment size, channel gradient, discharge, and underlying geologic structure.
- Understanding stream channel types helps explain landscape formation and erosion processes.
16.5 Review Questions
1. Are bedrock channels more likely to be found near the head or the mouth of a stream?
Bedrock channels are more likely to be found near the head of a stream, especially in the headwaters where streams have steep slopes. As streams flow downstream and encounter gentler gradients, they are more likely to transition to alluvial channels composed of sediment rather than cutting into solid rock.
2. Describe or sketch the evolution of a meander, including how an oxbow lake forms.
- Initially, a straight channel may develop a bend due to erosion on one bank and deposition on the opposite bank.
- Over time, the bend becomes more pronounced as erosion continues on the outer bank (cut bank) and sediment deposition occurs on the inner bank (point bar).
- As the bend migrates laterally downstream, it may eventually loop around to form an oxbow-shaped meander.
- During high-flow events, the neck of the meander may be breached, leading to the formation of a cutoff, isolating the oxbow-shaped segment from the main channel and forming an oxbow lake.
3. Describe a situation that might cause a stream channel to become braided.
A stream channel might become braided in situations where there is a high sediment supply, variable flow rates, and erodible bank material. For example:
- Glacial meltwater streams at the terminus of glaciers, where large volumes of sediment are delivered seasonally.
- Streams with highly variable discharge patterns, such as those in arid or semi-arid regions experiencing flash floods.
- Environments where there are abundant sediment sources, such as in areas with active erosion or deposition processes.
Stream Erosion (Notes from Instructor)
Lecture #12: Stream Erosion
Stream - A body of running water that is confined in a channel and moves downhill under the influence of gravity. A flood occurs when the stream fills and leaves its channel.
Channel - A long narrow depression eroded by the stream into rock or sediment.
Flood plain - A broad strip of land built up by sedimentation on either side of a stream channel.
Sheetwash - A thin layer of unchanneled water flowing downhill.
Discharge - The amount of volume of water that flows past a given point in a unit time.
Gradient - Downhill slope of the stream bed. Changes in gradient can cause deposition and erosion.
Runoff - The average annual rainfall on the area of the United States is equivalent to a layer of water 76 cm thick covering this same land surface. Of this layer, 45 cm returns to the atmosphere by evaporation and transpiration and 1 cm infiltrates the ground; the remaining 30 cm forms runoff, the portion of precipitation that flows over the land surface.
Base level - The limiting level below which a stream can not erode the land. As a stream flows downslope, its potential energy decreases and finally falls to zero as it reached the sea.
Types of Channels:
Meandering Channels - a channel which forms a series of smooth bends that are similar in size and resemble in shape the switch backs of a mountain road.
Meander cutoff - a new shorter channel across the narrow neck of a meander. Nearly 600 km of the Mississippi River channel has been abandoned since 1776 by meander cutoff. However, the river has not been shortened appreciably because the loss of channel due to cutoffs has been balanced by lengthening of the channel as other meanders have enlarged.
Oxbow lake - a lake within a cutoff meander.
Braided Channels - A stream which has lost its main channel by the deposition of bars. Usually such streams are heavily loaded. The stream widens continuously as more bars are deposited.
Stream's Load - Sediment load transported by a stream can be subdivided into bed load, suspended load, and dissolved load.
Bed Load - Heavy or large sediment particles that travel near or on the stream bed. These particles are either sand or gravel and they move by saltation (a series of short leaps or bounces of the bottom).
Suspended Load - Sediment that is light enough to remain lifted indefinitely above the bottom by water turbulence.
Dissolved Load - Soluble products of chemical weathering.
Stream Deposits:
Bar - a ridge of sediment, usually sand or gravel, deposited in the middle or along the banks of a stream.
Flood plain - part of a stream valley which is inundated by floodwater.
Levee - a broad, low ridge of fine alluvium build along the side of a channel by debris -laden flood water.
Terraces - the remnant of abandoned flood plains.
Alluvial Fan - a fan-shaped body of alluvium typically built where a stream leaves a steep mountain valley.
Deltas - a sedimentary deposit that forms where a stream flows into standing water and so-named because it may develop a crudely triangular shape that resembles the Greek letter delta.
Stream Patterns: - A tributary is a smaller stream joining the main stream at an acute angle. The nature by which tributaries join main streams determines the drainage pattern.
Dendritic - irregular branching of channels in many directions.
Trellised - Rectangular arrangement of channels in which principal tributary streams are parallel and very long.
Parallel - Parallel or subparallel channels that have formed on sloping surfaces underlain by homogeneous rocks.
Radial - channels radiate out, like the spokes of a wheel from a topographically high area.
Rectangular - channel system marked by right-angel bends.
16.6 Stream Valleys
Stream Valleys:
- Definition: Stream valleys consist of the channel and surrounding terrain that directs water into a stream, including the valley floor and walls.
- Characteristics: Vary from narrow, steep-sided valleys to wide, flat valleys; affected by stream erosion and weathering.
Valley Deepening:
- Description: Occurs when a stream's gradient is steep, resulting in downcutting and the formation of V-shaped valleys.
- Features: Rapids and waterfalls are prominent in V-shaped valleys, formed by variations in bedrock erodibility.
Valley Widening:
- Description: Occurs as streams approach graded conditions, leading to lateral erosion and meandering patterns.
- Features: Floodplains develop as streams widen their valleys, covering the valley floor with alluvium.
Incised Meanders and Stream Terraces:
- Incised Meanders: Meanders in steep, narrow bedrock valleys formed due to changes in base level, causing downcutting.
- Stream Terraces: Formed when a river adjusts to a relative drop in base level, leaving remnants of former floodplains as flat surfaces above newly forming floodplains.
Key Concepts:
- Base Level: Lowest elevation to which a stream can erode its channel; includes ultimate base level (sea level) and local base levels.
- Graded Streams: Streams with the necessary slope and characteristics to maintain sediment transport without erosion or deposition.
- Floodplain: Broad, flat valley floor covered with alluvium, inundated during flood stage.
- Erosional vs. Depositional Floodplains: Erosional floodplains form through lateral erosion, while depositional floodplains result from major fluctuations in conditions.
- Slot Canyons: Narrow valleys with vertical walls, often found in arid regions with resistant rock.
The base level upstream from the reservoir is raised, which reduces the stream’s flow velocity and leads to deposition and a reduced gradient.
A resistant layer of rock can act as a temporary base level. The stream concentrates its erosive energy on the resistant rock at the knickpoint. Eventually the river eliminates the knickpoint and reestablishes a smooth profile.
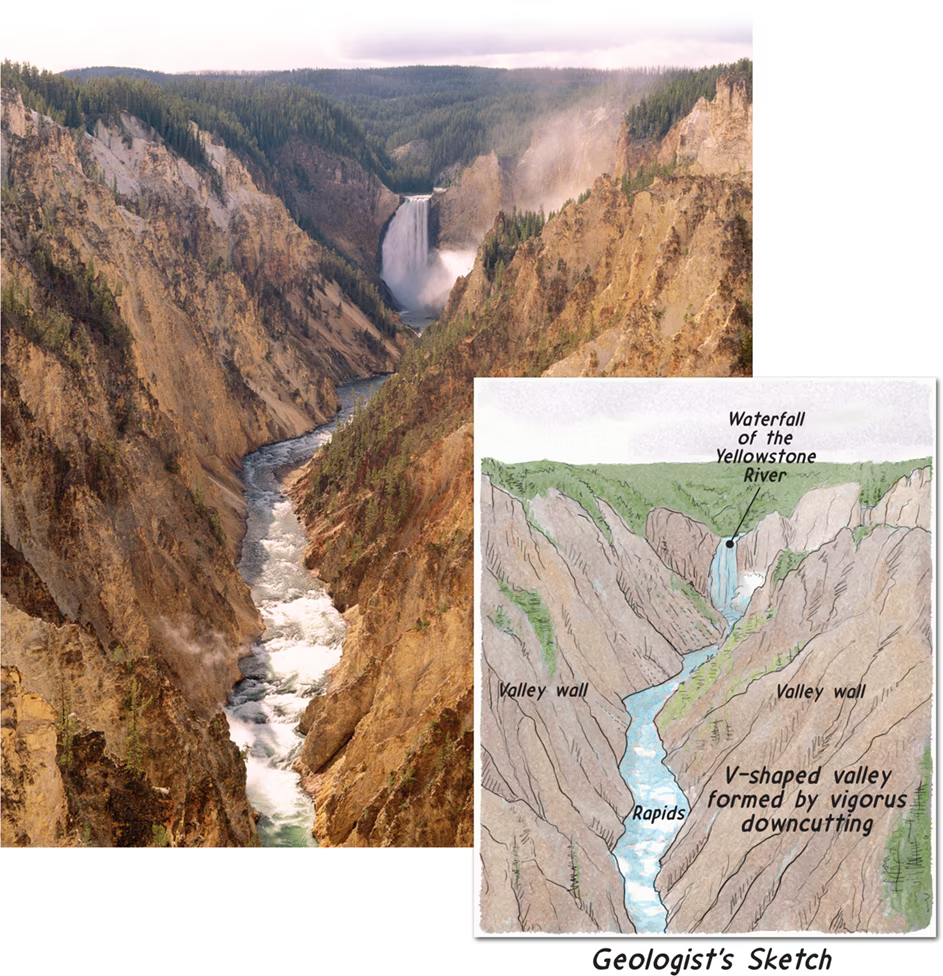
The V-shaped valley, rapids, and waterfalls indicate that the river is vigorously downcutting.
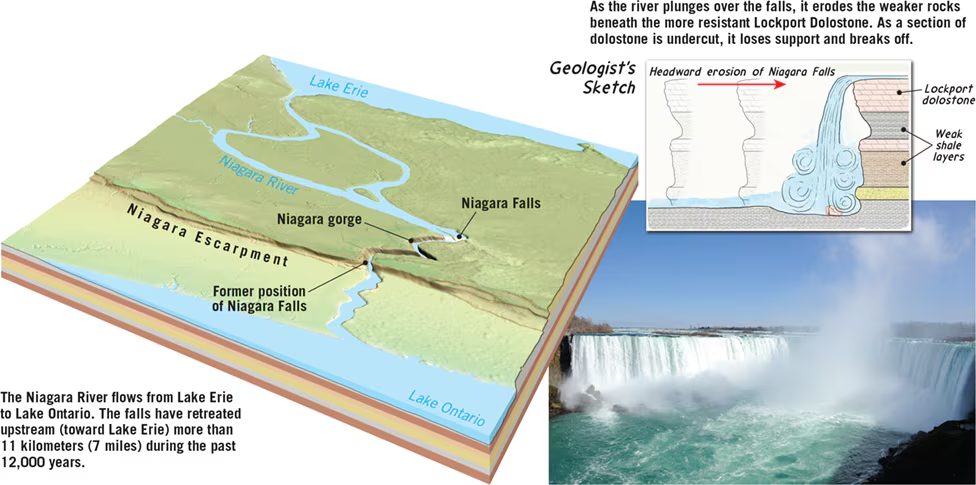
Continuous side-to-side erosion by shifting meanders gradually produces a broad, flat valley floor. Alluvium deposited during floods covers the valley floor.
16.6 Review Questions
1. Define base level and distinguish between ultimate base level and local, or temporary, base level.
- Question: What is base level, and how does it vary?
- Answer: Base level is the lowest point to which a stream can erode its channel. Ultimate base level refers to sea level, while local base level includes lakes, resistant rock layers, or other rivers acting as temporary limits to erosion.
2. Explain why V-shaped valleys often contain rapids and/or waterfalls.
- Question: What features are common in V-shaped valleys, and why?
- Answer: V-shaped valleys result from steep downcutting by streams. Rapids and waterfalls occur where streams encounter resistant rock layers, creating variations in gradient and flow velocity.
3. List two situations that would trigger the formation of incised meanders.
- Question: What conditions lead to the formation of incised meanders?
- Answer: Incised meanders form when there is a relative drop in base level due to either a drop in downstream base level or regional land uplift, causing streams to downcut and meander in narrow bedrock valleys.
16.7 Depositional Landforms
1. Deltas:
- Definition: Landforms formed at the mouth of rivers where sediment-laden streams enter still waters such as lakes, inland seas, or oceans.
- Formation Process:
- Sediments are deposited as the stream's forward motion slows upon entering the still water, forming foreset, topset, and bottomset beds.
- The delta grows outward as sediment accumulates, causing the stream's gradient to decrease and leading to the formation of distributaries.
- Deltas vary in shape and structure based on shoreline configurations, wave activity, and sediment supply.
- Example: The Mississippi River Delta, characterized by multiple subdeltas and a bird-foot delta shape.
2. Natural Levees:
- Definition: Elevated ridges parallel to river channels, formed by successive floods depositing coarse sediment adjacent to the channel.
- Formation Process:
- During floods, coarse sediments settle near the channel due to decreased flow velocity, forming elevated ridges.
- Over time, successive floods build up these ridges, creating natural levees that rise above the adjacent floodplain.
- Importance: Provide flood protection and influence drainage patterns, often leading to the formation of back swamps and yazoo tributaries.
3. Alluvial Fans:
- Definition: Fan-shaped deposits of sediment formed at the base of steep mountain slopes when high-gradient streams suddenly emerge onto flat plains.
- Formation Process:
- Streams deposit sediment rapidly due to the sudden decrease in velocity upon exiting narrow valleys, forming a cone- or fan-shaped accumulation.
- Coarser material settles near the apex of the fan, while finer material is carried toward the base.
- Example: Death Valley in California, known for its large alluvial fans formed by intermittent water flow during rainy periods.
Key Concepts:
- Deltas form at the mouths of rivers and are characterized by sediment deposition into still waters, creating distributaries and varying in shape based on sediment supply and wave activity.
- Natural levees are raised ridges parallel to river channels, formed by successive floods depositing coarse sediment adjacent to the channel.
- Alluvial fans develop at the bases of steep mountain slopes, where high-gradient streams deposit sediment rapidly upon exiting narrow valleys, forming cone- or fan-shaped accumulations.
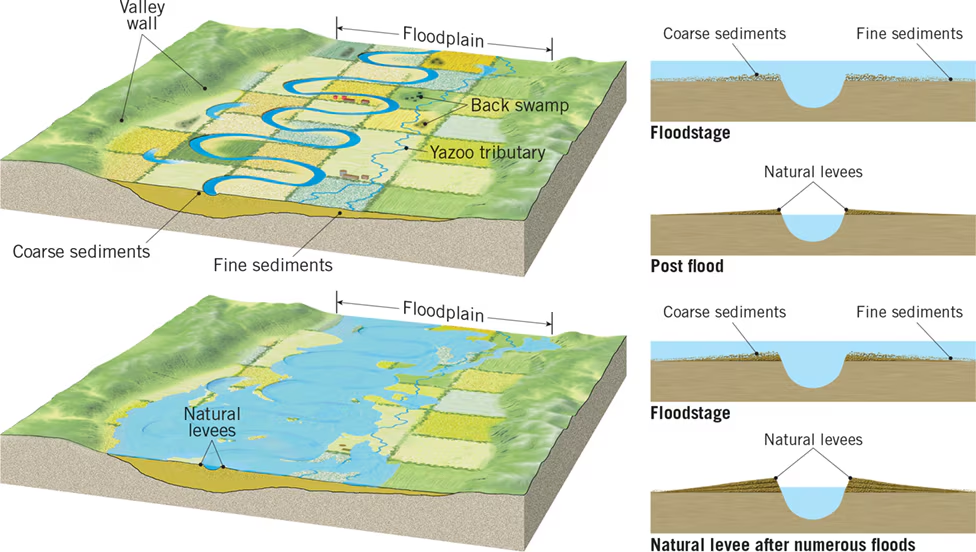
These gently sloping structures that parallel a river channel are created by repeated floods. Because the ground next to the channel is higher than the adjacent floodplain, back swamps and yazoo tributaries may develop.
Fans are deposited at the mouth of a valley that emerges from a mountainous or
upland area onto a relatively flat lowland. Usually coarse material is dropped near the apex of the fan, while finer materials are carried toward the base of the deposit. California’s Death Valley has many large fans. As adjacent fans grow larger, they may coalesce to form a steep apron of sediment called a bajada.
16.7 Review Questions
1. **Sketch a cross section of a simple delta and distinguish among the three types of beds that compose it.**
- **Question:** How would you sketch a cross section of a simple delta, and what are the three types of beds that make up its structure?
- **Answer:** A simple delta cross section consists of foreset beds (coarse particles from the bed load deposited downcurrent from the delta front), topset beds (deposited during flood stage), and bottomset beds (fine sediments settled away from the mouth in horizontal layers).
2. **Briefly describe the formation of a natural levee. How is this feature related to back swamps and yazoo tributaries?**
- **Question:** How do natural levees form, and what is their relationship to back swamps and yazoo tributaries?
- **Answer:** Natural levees form when successive floods deposit coarse sediment adjacent to a river channel during overflow, creating elevated ridges. Back swamps develop behind levees due to poor drainage, and yazoo tributaries flow through back swamps before rejoining the main river channel.
3. **Describe the formation of an alluvial fan.**
- **Question:** How does an alluvial fan form?
- **Answer:** An alluvial fan forms at the base of steep mountain slopes when high-gradient streams exit narrow valleys onto flat plains. The sudden decrease in velocity causes rapid sediment deposition, forming cone- or fan-shaped accumulations.
Chapter 17
17.1 The Importance of Groundwater
1. Groundwater as a Freshwater Source:
- Definition: Water stored beneath the Earth's surface in pore spaces and fractures.
- Importance:
- Represents the largest reservoir of readily available freshwater.
- Comprises about 30% of Earth's freshwater, excluding ice.
- Essential for sustaining human populations, agriculture, and ecosystems.
2. Geologic Role of Groundwater:
- Erosional Agent:
- Dissolves soluble rock, such as limestone, forming sinkholes and caverns.
- Equalizes Streamflow:
- Provides storage and sustains streamflow during dry periods by slowly releasing water to stream channels.
3. Groundwater as a Basic Resource:
- Usage:
- Supplies approximately 25% of freshwater in the United States.
- Widely used for irrigation, public/domestic, aquaculture, and industrial purposes.
- Advantages:
- Availability in areas lacking reliable surface water sources.
- Stored in subsurface pore spaces and fractures, providing a continuous supply.
4. Primary Uses of Groundwater:
- Irrigation: Largest use, supporting agriculture in arid regions.
- Public and Domestic: Indoor and outdoor household purposes, including drinking, cooking, bathing, and watering lawns.
- Aquaculture: Water for fish and shellfish farms.
- Industrial: Significant in mining, petroleum refining, and various manufacturing processes.
5. Trends in Water Use:
- Changing Demands: Growing population, increased industry, and agricultural needs.
- Conservation Efforts: Water use in the United States has decreased despite population growth, due to conservation and efficiency measures.
- Challenges: Some regions experiencing declining groundwater levels due to excessive extraction.
6. Environmental and Economic Importance:
- Ecosystem Support: Vital for maintaining habitats and biodiversity.
- Economic Impact: Essential for various industries and agricultural productivity.
- Conservation: Importance of sustainable management practices to ensure long-term availability.
7. Conclusion:
- Groundwater plays a critical role in sustaining life, ecosystems, and economies worldwide.
- Balancing human needs with environmental preservation is essential for the future of groundwater resources.
17.1 Review
- What percentage of Earth’s total freshwater supply is groundwater?
- Groundwater accounts for approximately 30% of Earth's total freshwater supply.
- What share of Earth’s liquid freshwater is groundwater?
- Groundwater represents approximately 96% of Earth's liquid freshwater.
- List two geologic roles that groundwater plays.
- Groundwater serves as an erosional agent by slowly dissolving soluble rock like limestone, forming features such as sinkholes and caverns.
- It helps equalize streamflow by providing sustained water flow during dry periods, thus contributing to the erosion and shaping of stream valleys.
- What share of U.S. freshwater is provided by groundwater?
- Groundwater provides about 25% of the total freshwater used in the United States.
- What is most groundwater used for?
- The majority of groundwater is used for irrigation purposes, particularly in agriculture.
17.2
1. Distribution of Water Beneath Earth's Surface:
- Rainfall paths: Some runoff, some evaporation/transpiration, some infiltration.
- Factors affecting infiltration: Slope steepness, surface material type, rainfall intensity, vegetation density and type.
- Zones:
- Soil Moisture Zone: Near-surface area where water is held by soil particles and used by plants.
- Zone of Saturation: Area where all spaces in sediment and rock are filled with water (groundwater).
- Capillary Fringe: Transitional zone above the water table where groundwater is held by surface tension.
- Unsaturated Zone: Area above the water table where pore spaces contain both air and water.
2. Zone of Saturation and Water Table:
- Zone of Saturation: Contains groundwater, where all pores in sediment and rock are filled with water.
- Water Table: Upper boundary of the zone of saturation; fluctuates due to factors like precipitation and pumping.
- Capillary Fringe: Layer above the water table where groundwater is drawn upward by capillary action.
- Unsaturated Zone: Area above the water table where soil pores contain air and some water.
3. Variations in the Water Table:
- Highly variable due to factors such as precipitation, geology, and human activities.
- Seasonal and yearly fluctuations depending on precipitation patterns.
- Monitored and mapped through well water levels, revealing irregular surface topography replication.
4. Factors Contributing to Water Table Variability:
- Groundwater Movement: Varies in rate, influencing water accumulation beneath high areas.
- Precipitation and Permeability: Varied precipitation and surface permeability lead to uneven water table levels.
- Human Activities: Pumping groundwater for irrigation or drinking can also alter the water table.
5. Interactions Between Groundwater and Streams:
- Gaining Streams: Receive water from groundwater inflow through streambeds.
- Losing Streams: Lose water to groundwater outflow through streambeds.
- Combined Interaction: Some sections of streams gain water, while others lose it.
- Groundwater Contribution to Streams: Groundwater is a major source of water for streams, influencing their flow and stability.
This detailed study guide covers the distribution of water beneath Earth's surface, characteristics of the zone of saturation and water table, variations in the water table, factors contributing to its variability, and interactions between groundwater and streams.
17.2 Review Questions
- When rain falls on land, what factors influence the amount of water that soaks in?
- Factors such as slope steepness, surface material type, rainfall intensity, and vegetation density and type influence the amount of water that infiltrates into the ground.
- Define groundwater and relate it to the water table.
- Groundwater is water that fills the spaces in sediment and rock beneath Earth's surface. It is closely related to the water table, which is the upper boundary of the zone of saturation where groundwater is found.
- A kitchen table is flat. Is this usually the case for a water table? Why?
- No, the water table is not usually flat like a kitchen table. It follows the topography of the land surface, meaning it is typically higher beneath hills and lower in valleys.
- Contrast a gaining stream and a losing stream.
- A gaining stream receives water from groundwater inflow through its streambed, while a losing stream loses water to the groundwater system through its streambed.
17.3 Storage and Movement of Groundwater
1. Factors Influencing Groundwater Storage and Movement:
- Porosity:
- Definition: Porosity refers to the percentage of the total volume of rock or sediment that consists of pore spaces.
- Influential Factors: Grain size, sorting, packing, and cementation affect porosity.
- Permeability:
- Definition: Permeability is a measure of a material's ability to transmit fluids and is crucial for groundwater movement.
- Influential Factors: Porosity alone does not indicate permeability; connectedness and size of pores are essential.
- Aquitards and Aquifers:
- Aquitards: Impermeable layers hinder groundwater movement.
- Aquifers: Permeable rock strata or sediments that transmit groundwater freely are termed aquifers.
2. Nature of Groundwater Movement:
- Slow Movement: Contrary to surface water, groundwater movement is slow, primarily occurring from pore to pore.
- Flow Paths: Groundwater moves along flow paths from recharge areas to discharge areas, such as springs, lakes, streams, and coastal areas.
3. Measuring Groundwater Movement:
- Henri Darcy's Experiments: In the mid-19th century, Darcy's experiments established principles of groundwater movement.
- Hydraulic Gradient: The slope of the water table determines groundwater velocity, expressed as the hydraulic gradient.
- Hydraulic Conductivity: Defined by Darcy, it represents the permeability of aquifers and influences flow rate.
- Darcy's Law: An equation used to determine discharge through an aquifer based on hydraulic gradient, hydraulic conductivity, and cross-sectional area.
4. Different Scales of Movement:
- Geographic Extent: Groundwater flow systems vary in size from local to regional scales.
- Flow Paths: Range from meters to kilometers, with complex interactions between shallow and deep systems.
- Cross-Sectional Diagram: Illustrates the complex arrangement of aquifers and aquitards, showing local and regional flow systems.
Key Points:
- Porosity and permeability are critical factors influencing groundwater storage and movement.
- Groundwater moves slowly from recharge to discharge areas along flow paths.
- Darcy's experiments laid the foundation for understanding groundwater movement, including hydraulic gradient and conductivity.
- Groundwater movement occurs at various scales, from local to regional, with complex interactions between different systems.
- Groundwater flows more rapidly through sediments having greater permeability than through materials having lower permeability. He defined a coefficient known as hydraulic conductivity that takes into account the permeability of the aquifer and the viscosity of the fluid. To determine discharge (Q)—that is, the actual volume of water that flows through an aquifer in a specified time—the following equation is used:where is the hydraulic gradient, K is the coefficient that represents hydraulic conductivity, and A is the cross-sectional area of the aquifer. This expression has come to be called Darcy’s law. Using this equation, if you know an aquifer’s hydraulic gradient, conductivity, and cross-sectional area, you can calculate its discharge.
Q= K A (H1-2)
d
The hydraulic gradient is determined by measuring the difference in elevation between two points on the water table divided by the distance between them, d. Wells are used to determine the height of the water table.
17.3 Review Questions
- Distinguish between porosity and permeability.
Porosity refers to the percentage of the total volume of rock or sediment that consists of pore spaces, while permeability is a measure of a material's ability to transmit fluids. Porosity indicates the storage capacity of groundwater, whereas permeability determines the ease with which water can flow through a material.
- What is the difference between an aquifer and an aquitard?
An aquifer is a permeable rock stratum or sediment that transmits groundwater freely, allowing significant water movement. Conversely, an aquitard is an impermeable layer that hinders or prevents water movement, acting as a barrier to groundwater flow.
- What factors cause water to follow the paths shown in Figure 17.10?
Water follows paths depicted in Figure 17.10 due to gravitational forces and pressure differentials. Groundwater moves from areas of higher elevation (recharge areas) to lower elevation (discharge areas), following the hydraulic gradient. Additionally, permeability variations in subsurface materials influence the direction and speed of groundwater movement.
- Relate groundwater movement to hydraulic gradient and hydraulic conductivity.
Groundwater movement is related to both hydraulic gradient and hydraulic conductivity. The hydraulic gradient, determined by the slope of the water table, governs the direction and velocity of groundwater flow. Hydraulic conductivity, a measure of a material's ability to transmit fluids, influences the rate of groundwater movement through porous media. Together, these factors determine how groundwater flows within an aquifer system.
17.4 Study Guide: Wells and Artesian Systems
- Wells:
- Definition: Wells are holes bored into the zone of saturation to extract groundwater, serving as small reservoirs for water migration and extraction.
- Significance: Groundwater from wells is a crucial source of drinking water, providing for approximately half the U.S. population and 96 percent of rural areas.
- Water Table and Wells:
- Fluctuations: The water table fluctuates seasonally, necessitating wells to penetrate below the water table for continuous water supply.
- Drawdown and Cone of Depression: Drawdown occurs when water is withdrawn from a well, creating a depression in the water table known as a cone of depression. This effect increases the hydraulic gradient near the well.
- Challenges of Well Drilling:
- Heterogeneous Subsurface Materials: Variability in subsurface materials leads to varying success rates in drilling wells. Perched water tables and geological structures impact the availability of groundwater.
- Artesian Systems:
- Definition: Artesian systems involve groundwater under pressure rising above the level of the aquifer, often leading to flowing artesian wells or springs.
- Conditions: Artesian systems require an inclined aquifer confined by impermeable layers (aquitards) above and below, creating confined aquifers.
- Flowing vs. Non-flowing Wells: Depending on the pressure surface's position relative to the ground level, wells in artesian systems can be flowing or non-flowing.
- Transmitting Water: Artesian systems act as conduits, transporting water over long distances from recharge areas to discharge points, influencing landscapes and ecosystems.
- Examples of Artesian Systems:
- Natural Springs: Artesian springs can emerge along natural fractures, contributing to oases in desert regions.
- Human Impact: Human activities, such as extensive well drilling, can deplete artesian systems, leading to decreased water flow and lower water tables.
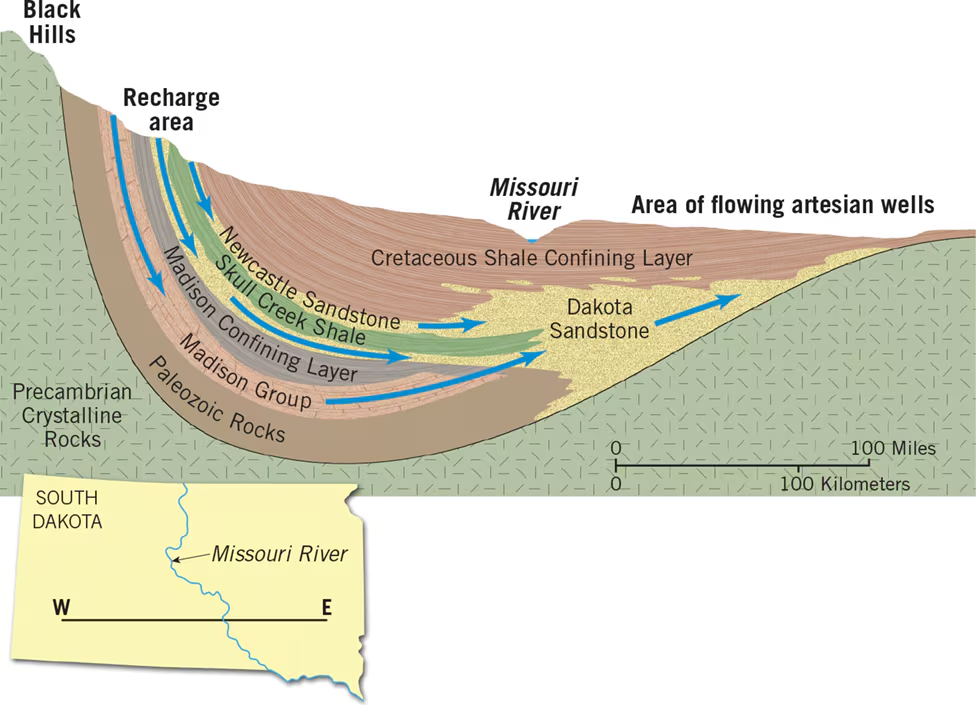
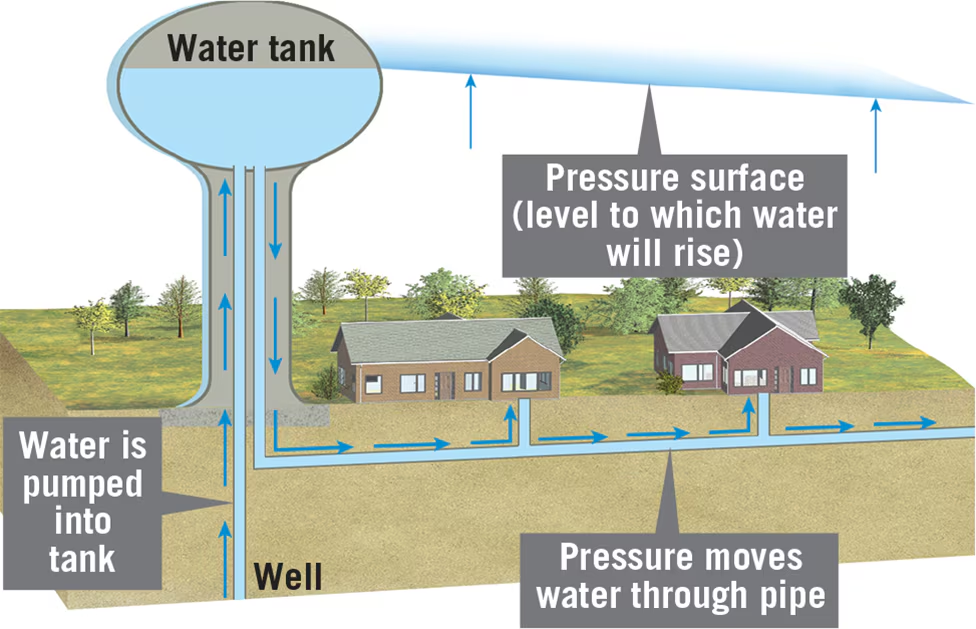
17.4 Review Questions
1. Define drawdown and relate this term to the term cone of depression.
- Drawdown: Drawdown refers to the lowering of the water table around a well when water is withdrawn, creating a depression in the water table.
- Relation to Cone of Depression: Drawdown contributes to the formation of a cone of depression, which is a roughly conical-shaped depression in the water table around a heavily pumped well. The drawdown decreases with distance from the well, resulting in a depression that resembles a cone.
2. Sketch a simple cross-section of an artesian system with a flowing well. Label aquitards, aquifers, and the pressure surface.
[Sketch]
- Aquitards: Impermeable layers above and below the aquifer.
- Aquifers: Permeable layers that transmit groundwater.
- Pressure Surface: Represents the level to which water would rise if not confined.
3. Why do some artesian wells not flow at Earth’s surface?
- Frictional Losses: Friction between the flowing water and the surrounding rock reduces the pressure, causing the water level to drop before reaching the surface.
- Hydraulic Gradient: Greater distance from the recharge area increases friction, resulting in less pressure and reduced flow at the surface.
- Depleted Reservoirs: Overuse of the aquifer can deplete the reservoir, lowering the water table and reducing pressure, leading to non-flowing artesian wells.
17.6 Environmental Problems Associated with Groundwater
- Overexploitation and Nonrenewable Resource Treatment:
- Groundwater is increasingly exploited, leading to overuse in some areas.
- In regions where withdrawal exceeds recharge, groundwater is essentially mined, causing long-term imbalances in the water table.
- Land Subsidence Due to Groundwater Withdrawal:
- Excessive pumping causes the ground to sink as the weight of overlying sediments compresses due to decreased water pressure.
- Examples include the San Joaquin Valley in California, southern Arizona, Las Vegas, New Orleans, and Houston.
- Impact of Prolonged Drought:
- Drought increases groundwater usage, leading to land subsidence.
- California's severe drought in 2016 resulted in significant land subsidence due to increased groundwater pumping.
- Saltwater Intrusion:
- Coastal areas face the threat of saltwater encroachment into freshwater aquifers.
- Over-pumping lowers the water table, allowing saltwater to intrude into wells, contaminating freshwater supplies.
- Groundwater Contamination:
- Pollution from sewage, septic tanks, broken sewer systems, and farm wastes threatens groundwater quality.
- Hazardous chemicals, fertilizers, pesticides, and industrial materials also contribute to contamination.
- Slow groundwater movement can delay detection of pollution until after drinking water is affected.
- Prevention is the most effective solution to groundwater contamination.
Important Details:
- High Plains Aquifer: Example of severe groundwater depletion due to intense irrigation and limited recharge.
- Land Subsidence Examples: San Joaquin Valley in California, southern Arizona, Las Vegas, New Orleans, and Houston.
- Saltwater Intrusion: Occurs in coastal areas where over-pumping allows saltwater to contaminate freshwater aquifers.
- Impact of Prolonged Drought: California's 2016 drought led to increased groundwater pumping and land subsidence.
- Groundwater Contamination Sources: Sewage, septic tanks, broken sewer systems, farm wastes, highway salt, fertilizers, pesticides, industrial chemicals, and landfill leachate.
- Solutions: Prevention is crucial. Other solutions include abandoning contaminated water supplies, natural recharge, and pumping and treating polluted water.
17.6 Review Questions
- Describe the problem associated with pumping groundwater for irrigation in the southern High Plains.
- The problem in the southern High Plains arises from intense irrigation, primarily for agriculture, leading to excessive groundwater withdrawal. The area receives limited precipitation, resulting in minimal recharge to replenish the aquifer. As a consequence, groundwater levels decline rapidly due to overuse, risking depletion of the aquifer and posing a threat to sustainable water supply.
- Explain why the ground may subside after groundwater is pumped to the surface.
- Ground subsidence occurs when groundwater is pumped from wells at a faster rate than natural recharge processes can replenish it. As water is withdrawn, the water pressure decreases, causing the overlying sediment to compact. This compaction results in a loss of support, leading to the sinking or subsidence of the ground surface.
- Which aquifer would be most effective in purifying polluted groundwater: coarse gravel, sand, or cavernous limestone?
- The aquifer composed of cavernous limestone would be the most effective in purifying polluted groundwater. Cavernous limestone contains numerous interconnected openings and conduits, allowing groundwater to flow slowly through the aquifer. This slow flow rate provides ample time for natural processes such as mechanical filtration, chemical oxidation, and assimilation by other organisms to purify the contaminated water.
- Describe a significant problem that may arise when groundwater is heavily pumped at a coastal site.
- A significant problem that may arise when groundwater is heavily pumped at a coastal site is saltwater intrusion. Over-pumping of groundwater lowers the water table, creating a hydraulic gradient that draws saltwater from the ocean into freshwater aquifers. As a result, the intrusion of saltwater contaminates the freshwater supply, rendering it unsuitable for consumption or agricultural use. This phenomenon poses a severe threat to coastal communities dependent on groundwater for their water needs.
17.7 Geologic Work of Ground Water
Definitions:
- Caverns: Large underground chambers formed by the dissolution of soluble rocks such as limestone by groundwater.
- Karst Topography: A landscape characterized by sinkholes, caves, and underground drainage systems, formed due to the dissolution of soluble rocks like limestone by groundwater.
Important Details:
- Formation of Caverns and Development of Karst Topography:
- Groundwater dissolves soluble rocks, particularly limestone, forming caverns and contributing to the development of karst topography.
- Caverns are created by the dissolution of limestone by acidic groundwater, mainly in the zone of saturation.
- Cavern development occurs along lines of weakness in the rock, gradually enlarging cavities into interconnected passages.
- Dripstone formations, including stalactites, stalagmites, and columns, are depositional features formed by the precipitation of calcium carbonate from dripping water in caves.
- Karst Topography:
- Karst topography is characterized by irregular terrain with sinkholes, caves, and underground drainage systems.
- It occurs in areas underlain by soluble rocks like limestone, with notable examples in Kentucky, Tennessee, and Florida.
- Sinkholes are depressions formed either gradually by the dissolution of limestone or abruptly by the collapse of cavern roofs.
- Karst regions typically lack surface drainage, with runoff funneled underground through sinkholes.
- Tower Karst:
- Tower karst is a type of karst topography found in wet tropical and subtropical regions, characterized by steep-sided hills or towers rising abruptly from the ground.
- It forms in areas with thick beds of highly jointed limestone, where groundwater dissolves large volumes of rock, leaving behind residual towers.
- Examples of tower karst regions include southern China, Puerto Rico, and western Cuba.
Key Takeaways:
- Groundwater plays a significant role in shaping the Earth's surface through the dissolution of soluble rocks, leading to the formation of caverns and karst topography.
- Caverns exhibit unique dripstone formations created by the deposition of calcium carbonate from dripping water.
- Karst topography is characterized by sinkholes, caves, and underground drainage systems, with variations such as tower karst found in tropical regions.
17.7 Review Questions
How does groundwater create caverns?
- Groundwater creates caverns through a process of dissolution. When groundwater, often containing carbonic acid, comes into contact with soluble rocks like limestone, it reacts with the rock, dissolving it over time. This erosion process enlarges small cracks and fissures in the rock, gradually forming larger cavities underground.
What causes cavern formation to stop at one level (depth) but continue or begin at a lower level?
- Cavern formation often stops at one level due to changes in the water table. As streams erode deeper valleys, the water table drops, causing the groundwater level to decrease. Caverns that were forming at or just below the previous water table level may be abandoned as the water drains away. However, cavern formation can continue or begin at a lower level if the water table drops further, exposing new areas of soluble rock to the erosive action of groundwater.
How do stalactites and stalagmites form?
- Stalactites and stalagmites form in caves through the deposition of minerals carried by dripping water. Water containing dissolved calcium carbonate drips from the ceiling of a cave, leaving behind small amounts of calcite as it evaporates. Over time, these deposits accumulate, forming icicle-like structures hanging from the ceiling (stalactites) or cone-shaped mounds rising from the floor (stalagmites). Stalactites form from the ceiling downward, while stalagmites form from the floor upward.
Describe two ways in which sinkholes form.
Sinkholes can form gradually or abruptly. Gradual sinkhole formation occurs over time as rainwater, charged with carbon dioxide, seeps through the soil and dissolves the underlying limestone or other soluble rock. The bedrock surface gradually subsides, and the soil above is carried away by groundwater flow, creating shallow depressions.
- Abrupt sinkhole formation occurs when the roof of an underground cavern collapses under its own weight. This sudden collapse creates steep-sided and deep sinkholes. Such collapses can pose serious hazards, especially in populated areas.
Chapter 13
13.1 p. 365 An Emerging Picture of the Ocean Floor
1. Bathymetry:
- Definition: Bathymetry refers to the measurement of ocean depths and the mapping of the ocean floor's topography.
- Historical Perspective: Early techniques involved laboriously lowering a weighted line overboard and recording depths. The HMS Challenger's historic voyage (1872-1876) marked the first comprehensive study of the global ocean, where water depths were measured manually.
- Modern Techniques:
- Echo Sounders: Developed in the early 20th century, echo sounders transmit sound pulses (pings) into the water, measuring the time taken for the echo to return. This data is used to calculate water depth.
- Sidescan Sonar: Developed post-World War II, sidescan sonar instruments send out sound waves perpendicular to a ship's path, providing image-like representations of the seafloor.
- Multibeam Sonar: High-resolution multibeam sonar instruments, developed in the 1990s, use multiple sound beams to map wide sections of the ocean floor with detailed depth information.
2. Mapping the Ocean Floor from Space:
- Satellite Altimetry: Satellites equipped with radar altimeters measure variations in sea-surface elevation caused by gravitational forces exerted by seafloor features. This data, combined with traditional sonar measurements, aids in creating large-scale maps of the ocean floor.
3. Provinces of the Ocean Floor:
- Continental Margins: The submerged outer edge of a continent, consisting of the continental shelf, slope, and rise.
- Deep-Ocean Basins: Large areas of the seafloor that lie beyond the continental margins, characterized by abyssal plains, deep-sea trenches, and seamounts.
- Oceanic (Mid-Ocean) Ridges: Underwater mountain ranges formed by tectonic activity where new oceanic crust is created.
Key Points:
- The HMS Challenger's voyage marked the beginning of systematic bathymetric surveys.
- Modern techniques like multibeam sonar allow for high-resolution mapping of the ocean floor.
- Satellite altimetry complements traditional sonar measurements, aiding in understanding large-scale features.
- The ocean floor is divided into continental margins, deep-ocean basins, and oceanic ridges, each with distinct geological characteristics.
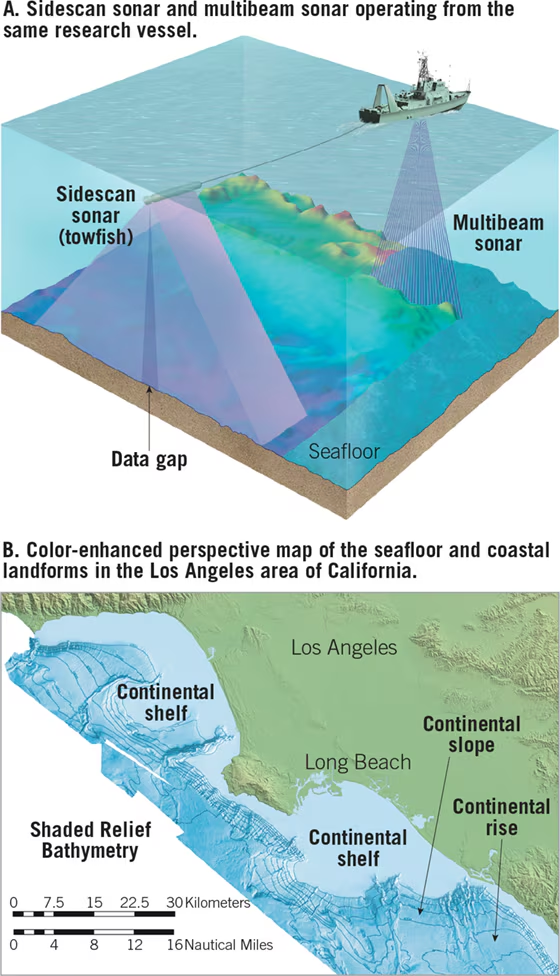
13.1 Review
- Define bathymetry: Bathymetry refers to the measurement of ocean depths and the mapping of the ocean floor's topography.
- Describe how satellites orbiting Earth can determine features on the seafloor without being able to directly observe them beneath several kilometers of seawater:
Satellites equipped with radar altimeters can measure variations in sea-surface elevation caused by gravitational forces exerted by seafloor features. By bouncing microwaves off the sea surface and compensating for factors like waves, tides, currents, and atmospheric effects, satellites can detect subtle differences in sea surface height. These variations are indicative of the underlying topography of the ocean floor. By combining this data with traditional sonar measurements, which provide direct information about the ocean floor's depth, satellites contribute to the creation of large-scale maps of the ocean floor, even though they cannot directly observe features beneath several kilometers of seawater.
- List the three major provinces of the ocean floor:
The three major provinces of the ocean floor are:
- Continental Margins
- Deep-Ocean Basins
- Oceanic (Mid-Ocean) Ridges
13.2 Continental Margins
1. Continental Margins:
- Definition: Continental margins are the outer edges of the continents where continental crust transitions to oceanic crust.
- Types of Continental Margins: Two types of continental margins exist: passive and active.
2. Passive Continental Margins:
- Definition: Passive continental margins are geologically inactive regions located far from plate boundaries.
- Major Features:
- Continental Shelf: Gently sloping, submerged surface extending from the shoreline toward the ocean basin, consisting mainly of continental crust capped with sedimentary rocks and sediments.
- Continental Slope: Relatively steep zone marking the boundary between continental crust and oceanic crust.
- Continental Rise: Gradual incline seaward from the continental slope, consisting of a thick accumulation of sediment moved down from the continental slope.
3. Active Continental Margins:
- Definition: Active continental margins are located along convergent plate boundaries where oceanic lithosphere is being subducted beneath the leading edge of a continent.
- Major Features:
- Deep-Ocean Trenches: Deep, narrow furrows formed at convergent plate boundaries where subduction occurs.
- Accretionary Wedge: Accumulation of deformed sediment and scraps of oceanic crust plastered against the edge of the overriding plate.
- Subduction Erosion: Process where sediment and rock are scraped off the bottom of the overriding plate and transported into the mantle by the subducting plate.
Key Points:
- Passive Continental Margins: Geologically inactive regions, characterized by wide continental shelves and accumulation of sediments.
- Active Continental Margins: Located at convergent plate boundaries, associated with tectonic activity such as subduction, deep-ocean trenches, and accretionary wedges.
- Distinct Features: The major features of continental margins include continental shelves, slopes, rises, deep-ocean trenches, accretionary wedges, and subduction erosion.
Distribution of subduction zones: Ring of Fire
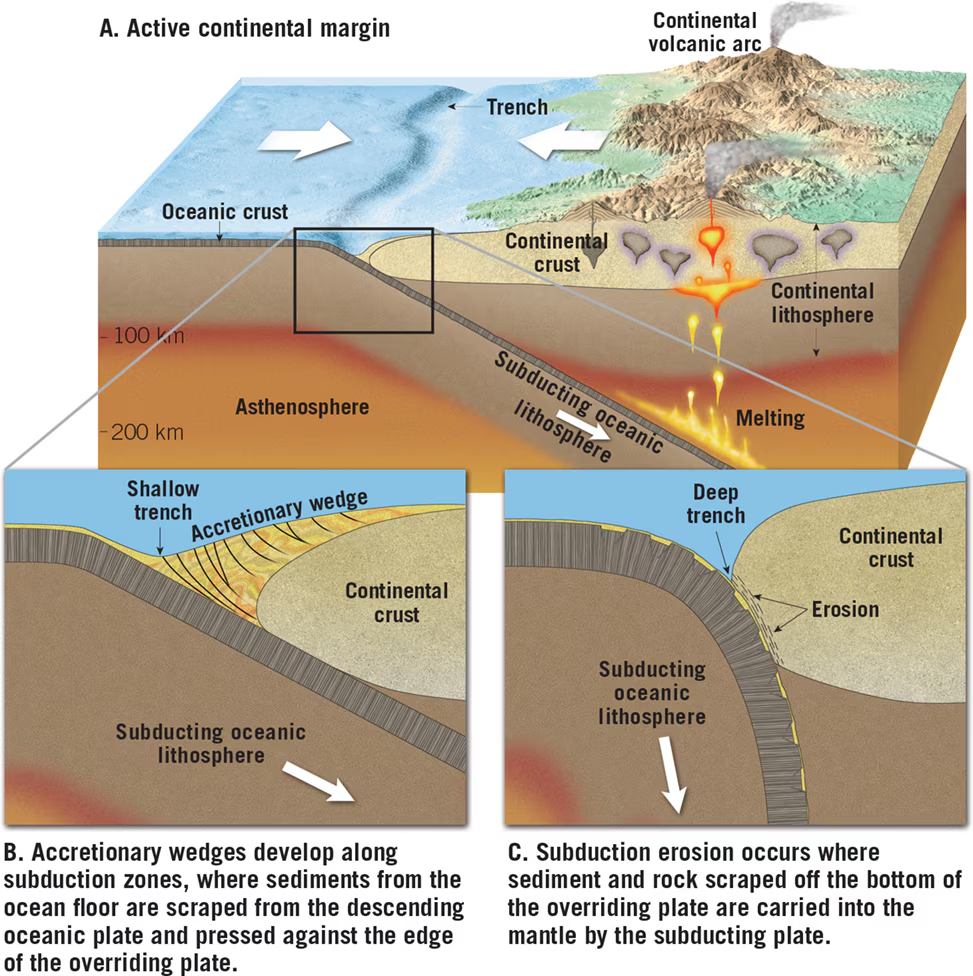
13.2 Review Questions
- List the three major features of a passive continental margin:
- Continental Shelf
- Continental Slope
- Continental Rise
- Describe the differences between active and passive continental margins. Where is each type found?
- Passive Continental Margins:
- Definition: Geologically inactive regions located far from plate boundaries.
- Major Features: Wide continental shelves, gentle continental slopes, and gradual continental rises.
- Location: Found along coastlines away from plate boundaries, such as the Atlantic coast of North America.
- Active Continental Margins:
- Definition: Located at convergent plate boundaries where oceanic lithosphere is being subducted beneath the leading edge of a continent.
- Major Features: Deep-ocean trenches, accretionary wedges, and subduction erosion features.
- Location: Typically found bordering the Pacific Ocean, where subduction zones are prevalent.
- Passive Continental Margins:
- How are active continental margins related to plate tectonics?
- Active continental margins are closely related to plate tectonics, specifically the process of subduction. At these margins, oceanic lithosphere is being subducted beneath continental plates, leading to tectonic activity such as the formation of deep-ocean trenches, accretionary wedges, and subduction erosion features. The movement and interaction of tectonic plates drive the geological processes observed at active continental margins, highlighting the connection between plate tectonics and the Earth's surface features.
13.3 Features of Deep-Ocean Basins p.371
1. Major Features of Deep-Ocean Basins:
- Deep-Ocean Trenches:
- Long, relatively narrow troughs, often the deepest parts of the ocean.
- Typically found along the margins of the Pacific Ocean.
- Example: Challenger Deep in the Mariana Trench.
- Abyssal Plains:
- Deep, incredibly flat features, the most level places on Earth.
- Covered by thick accumulations of sediment, primarily consisting of fine sediments from land, mineral matter precipitated out of seawater, and shells/skeletons of marine organisms.
- Volcanic Structures:
- Seamounts and Volcanic Islands:
- Submarine volcanoes rising hundreds of meters above the surrounding seafloor.
- Some grow large enough to become oceanic islands.
- Guyots:
- Submerged, flat-topped seamounts formed by inactive volcanic islands.
- Oceanic Plateaus:
- Massive structures resembling lava plateaus composed of flood basalts.
- Generated from vast outpourings of fluid basaltic lavas.
- Seamounts and Volcanic Islands:
2. Darwin's Hypothesis on Coral Atolls:
- Charles Darwin's hypothesis explains the origin of ring-shaped coral atolls.
- Darwin proposed that volcanic islands gradually sink over time, and corals respond by building the reef complex upward.
- Atolls may eventually become guyots when the rate of subsidence exceeds the ability of corals to build up the reef or when reef-building organisms die out.
Key Points:
- Deep-Ocean Trenches: Formed at plate boundaries where oceanic lithosphere subducts, associated with volcanic activity and earthquake generation.
- Abyssal Plains: Covered by thick accumulations of sediment, primarily composed of fine sediments, mineral matter, and marine organisms' remains.
- Volcanic Structures: Include seamounts, volcanic islands, guyots, and oceanic plateaus, formed by underwater volcanic activity and processes.
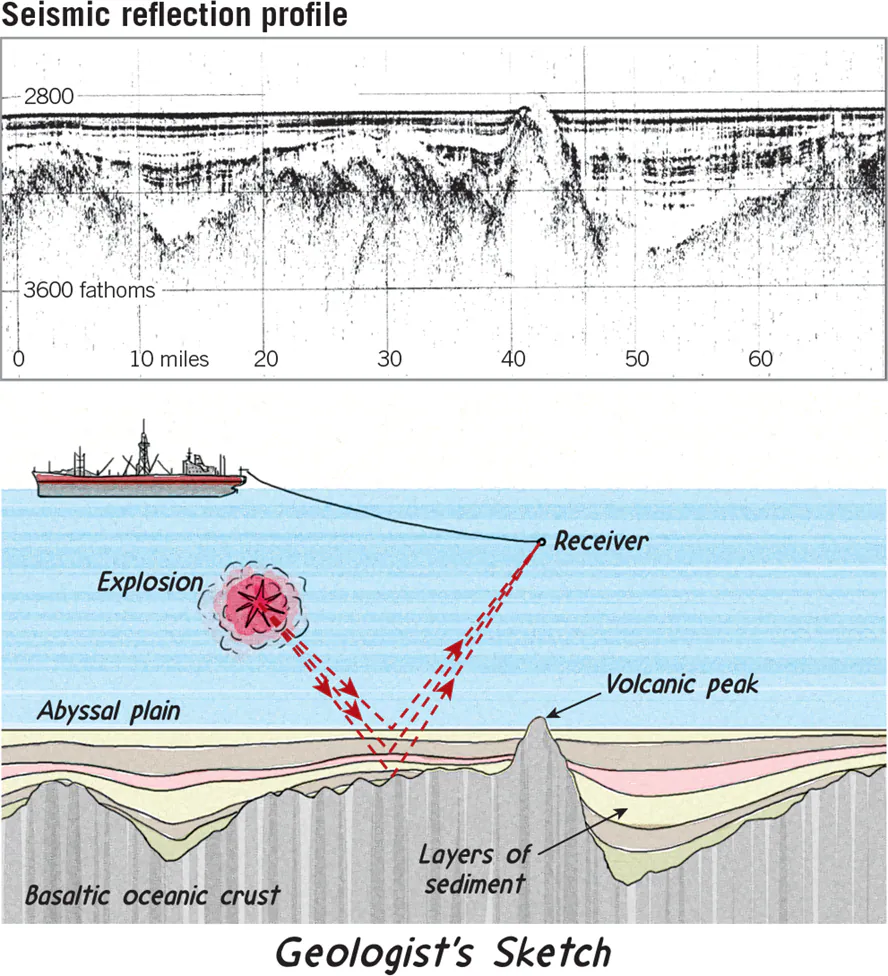
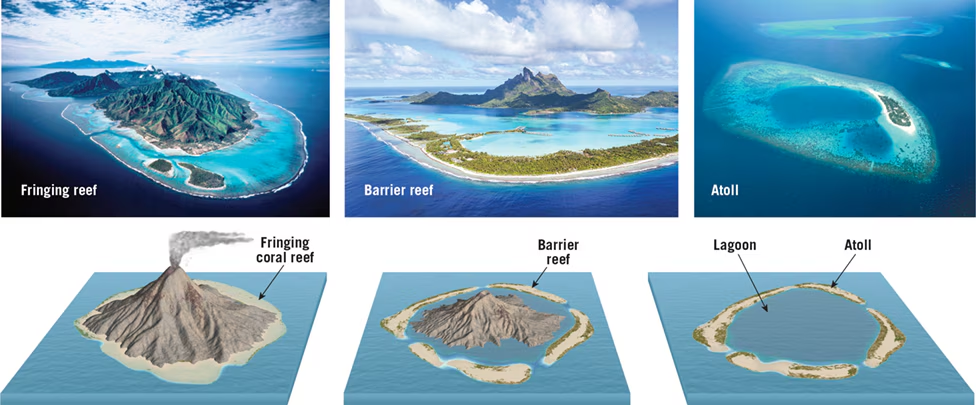
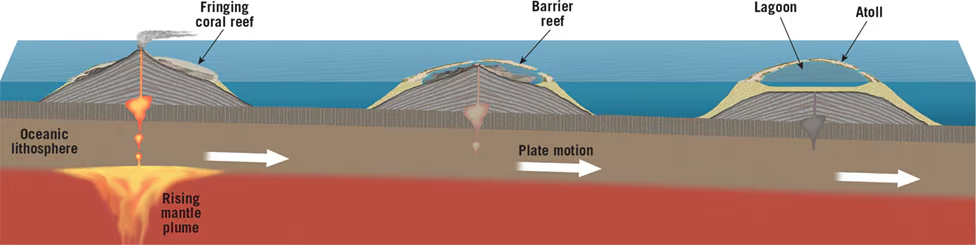
13.3 Review Questions
1. Explain how deep-ocean trenches are related to convergent plate boundaries.
- Answer: Deep-ocean trenches are formed at convergent plate boundaries where oceanic lithosphere is being subducted beneath the leading edge of a continent or another oceanic plate. Trenches are sites of plate convergence where slabs of oceanic lithosphere subduct and plunge back into the mantle. This process of subduction leads to the formation of deep, narrow furrows known as deep-ocean trenches.
2. Why are abyssal plains more extensive on the floor of the Atlantic than on the floor of the Pacific?
- Answer: Abyssal plains are more extensive on the floor of the Atlantic than on the floor of the Pacific because the Atlantic Ocean has fewer trenches to act as traps for sediment carried down the continental slope. Abyssal plains owe their relatively featureless topography to thick accumulations of sediment that have buried an otherwise rugged ocean floor. With fewer trenches in the Atlantic to trap sediment, the accumulation of sediment is more extensive, resulting in more extensive abyssal plains.
3. How does a flat-topped seamount, called a guyot, form?
- Answer: Flat-topped seamounts, known as guyots, form when inactive volcanic islands gradually sink and disappear below the water surface. During their existence, inactive volcanic islands are gradually lowered to near sea level by weathering and erosion. As a moving plate carries inactive volcanic islands away from the elevated oceanic ridge or hot spot over which they formed, they gradually sink. Submerged, flat-topped seamounts that formed in this manner are called guyots.
4. Using Darwin’s hypothesis, place these coral reefs in order from youngest to oldest: barrier reef, atoll, and fringing reef.
- Answer: According to Darwin's hypothesis, coral reefs progress in the following order from youngest to oldest:
- Fringing Reef: Forms along the margins of a volcano.
- Barrier Reef: Develops with a volcano in the middle, creating a lagoon between the reef and the shore.
- Atoll: Consists of a continuous or broken ring of coral reef surrounding a central lagoon, indicating the sinking of the volcano beneath the sea level and subsequent reef growth.
Chapter 18
18.1 Ocean Waves and Shoreline Dynamics
1. Deep-Ocean Trenches and Convergent Plate Boundaries
- Definition: Deep-ocean trenches are long, narrow furrows formed at convergent plate boundaries where oceanic lithosphere is being subducted beneath another plate.
- Details: Trenches are sites of plate convergence where oceanic plates plunge back into the mantle, generating deep depressions in the ocean floor.
2. Abyssal Plains and Oceanic Floors
- Definition: Abyssal plains are extensive, flat regions on the ocean floor.
- Details: They are more extensive in the Atlantic Ocean due to fewer trenches, allowing sediment accumulation. Sediment accumulation buries rugged ocean floor, creating flat plains.
3. Formation of Guyots
- Definition: Guyots are flat-topped seamounts formed from inactive volcanic islands.
- Details: Inactive volcanic islands gradually sink below sea level due to erosion and plate motion, resulting in submerged flat-topped seamounts known as guyots.
4. Coral Reef Development According to Darwin's Hypothesis
- Definition: Coral reefs include fringing reefs, barrier reefs, and atolls.
- Details: According to Darwin, coral reefs progress from fringing reefs (youngest), forming along volcanic margins, to barrier reefs (middle-aged), with a central lagoon, and finally atolls (oldest), representing submerged volcanic islands with surrounding coral reefs.
5. Shoreline Dynamics
- Definition: The shoreline is the dynamic interface where land and sea meet.
- Details: It undergoes constant modification by waves, erosion, and sediment transport, exhibiting a complex character influenced by various geologic processes.
6. Ocean Wave Characteristics
- Definition: Ocean waves are generated by wind and carry energy across the sea surface.
- Details: Wave characteristics include wave height, wavelength, and wave period, influenced by wind speed, duration, and fetch. Waves transform into swells and travel great distances across ocean basins.
7. Circular Orbital Motion of Waves
- Definition: Circular orbital motion describes the movement of water particles within waves.
- Details: Water particles move in circular orbits as waves travel forward, with diminishing motion with depth. Circular motion allows energy transfer through water without significant forward movement.
8. Wave Behavior Near Shore
- Definition: Wave behavior changes as waves approach the shore.
- Details: As waves encounter shallow water, their speed decreases, wavelength shortens, and wave height increases. Eventually, waves break, forming surf, and water rushes up the shore as swash, then back down as backwash.
When water depth is less than half the wavelength, wave speed decreases, and the faster-moving waves farther from shore begin to catch up, causing the distance between waves (the wavelength) to decrease. This causes an increase in wave height, to the point where the waves finally pitch forward and break in the surf zone. The first portion of the animation illustrates the ideas presented in this figure.
20.1 Question Review p.563
1. Why is the shoreline described as being an interface?
Answer: The shoreline is described as an interface because it marks the dynamic boundary where land, air, and sea interact. It's a zone where the ocean's water meets the land, resulting in constant changes and interactions between these different elements.
2. List three factors that determine the height, length, and period of a wave.
Answer:
- Wind Speed: Faster winds generate larger waves.
- Duration of Wind: Longer durations result in larger waves.
- Fetch: The distance over which the wind blows across the water's surface determines wave size.
3. Describe the motion of a floating object as a wave passes.
Answer: As a wave passes, a floating object moves in a circular orbital motion. It rises as the crest approaches, moves forward as the crest passes, descends as the trough approaches, and moves backward as the trough passes. This movement results in a circular path for the floating object.
4. How do a wave’s speed, wavelength, and height change as the wave moves into shallow water and breaks?
Answer:
- Wave Speed: Wave speed decreases as the wave moves into shallow water due to interaction with the seafloor.
- Wavelength: Wavelength shortens as the wave approaches the shore, causing waves to become closer together.
- Wave Height: Wave height increases as the wave enters shallow water and eventually breaks. Breaking waves result in higher wave heights due to the compression of energy as the wave front collapses.
20.2 Beaches and Shoreline Processes
Definitions:
- Beach: An accumulation of sediment found along the landward margin of a water body, composed of locally abundant materials such as sand, gravel, or shell fragments.
- Wave Erosion: The process by which waves impact, pressure, and abrasion erode the shoreline, cliffs, and other coastal features.
- Wave Refraction: The bending of waves as they approach the shore, affecting the distribution of energy along the shoreline and influencing erosion, sediment transport, and deposition.
Important Details:
- Wave Erosion and Sediment Movement:
- Waves erode the shoreline through impact, pressure, and abrasion during storms.
- Abrasion is intensified in the surf zone, leading to the smoothing and rounding of rocks along the shore.
- Sediment on the beach is in constant motion due to wave action, leading to the transport of sand and other materials along the shoreline.
- Sand Movement on the Beach:
- Wave energy moves sand along the beach face and in the surf zone, both parallel and perpendicular to the shoreline.
- Swash and backwash movements determine the direction of sand movement on the beach.
- Beaches experience seasonal changes in width due to variations in wave energy, with wide beaches forming during calm periods and narrower beaches during stormy periods.
- Wave Refraction:
- Wave refraction causes waves to bend as they approach shallow water and align nearly parallel to the shoreline.
- Differential wave attack along irregular coastlines results in erosion of headlands and sediment deposition in bays, leading to shoreline straightening over time.
- Longshore Transport:
- Beach drift and longshore currents transport sediment in a zigzag pattern along the beach face.
- Longshore currents move substantially more sediment than beach drift and can transport large quantities of sand and gravel along the shore.
- Longshore currents flow southward along both the Atlantic and Pacific shores of the United States.
- Rip Currents:
- Rip currents are concentrated movements of water flowing opposite to breaking waves, often recognized by their interference with incoming waves.
- Rip currents can be hazardous to swimmers, carrying them away from the shore, and the best strategy for exiting a rip current is to swim parallel to the shore for a few tens of meters.
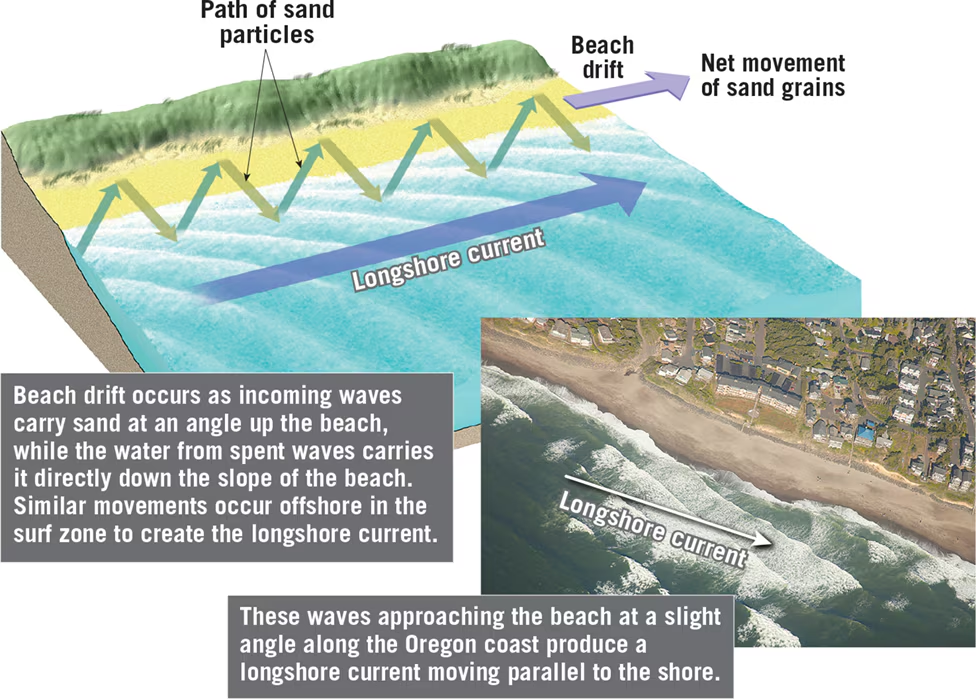
20.2 Review Questions
- Why do waves approaching the shoreline often bend?
Waves approaching the shoreline often bend due to wave refraction. This bending occurs because the part of the wave nearest the shore reaches shallow water and slows first, whereas the end that is still in deep water continues forward at its full speed. As a result, the wave front may approach nearly parallel to the shore, regardless of the original direction of the wave.
- What is the effect of wave refraction along an irregular coastline?
Wave refraction along an irregular coastline affects the distribution of wave energy along the shore, leading to differential wave attack. Headlands that project into the water experience concentrated wave impact from waves bending around them, causing erosion. In contrast, bays experience weakened wave activity due to wave divergence, leading to sediment accumulation and beach formation. Over time, wave refraction can contribute to shoreline straightening as erosion occurs in headlands and sediment deposition occurs in bays.
- Describe the two processes that contribute to longshore transport.
Longshore transport involves two main processes:
- Beach Drift: Beach drift occurs when the uprush of water from breaking waves (swash) moves sediment obliquely along the beach face. This process transports sand and pebbles parallel to the shoreline, with the swash carrying sediment up the beach face and the backwash returning sediment down the beach slope.
- Longshore Currents: Longshore currents are generated by waves approaching the beach at an angle. These currents flow parallel to the shoreline within the surf zone and move substantially more sediment than beach drift. Longshore currents transport fine suspended sand and roll larger sand and gravel along the bottom, contributing to the movement of sediment along the shore.
20.3 Shoreline Features
Definitions:
- Erosional Features: Landforms along coastlines formed primarily by erosional processes.
- Depositional Features: Coastal features created by the deposition of sediment transported by various processes.
Important Details:
- Erosional Features:
- Wave-Cut Cliffs: Formed by the cutting action of surf against the base of coastal land, leading to the retreat of the cliff.
- Wave-Cut Platforms: Flat, benchlike surfaces left behind by the receding cliff as erosion progresses.
- Marine Terraces: Wave-cut platforms uplifted above sea level by tectonic forces, recognized by gentle seaward-sloping shape.
- Sea Arches and Sea Stacks: Result from vigorous wave attack on headlands, with sea arches forming initially and sea stacks as isolated remnants.
- Depositional Features:
- Spits: Elongated ridges of sand projecting from the land into the mouth of an adjacent bay, often with a hooked end.
- Baymouth Bars: Sandbars completely crossing a bay, sealing it off from the open ocean.
- Tombolos: Sand ridges connecting an island to the mainland or to another island, formed similarly to spits.
- Barrier Islands: Low ridges of land parallel to the coast, located offshore and separated from the mainland by lagoons.
- Barrier Islands:
- Formed through various processes, including spits severed from the mainland, accumulation of sand from turbulent waters, and former sand dune ridges flooded by rising sea levels.
- Characterized by sand dunes, lagoons, and coastal marshes, providing protection to the mainland from storm waves.
- The Evolving Shore:
- Shorelines undergo continuous modification due to erosional and depositional processes.
- Irregular coastlines may become straighter and more regular over time due to marine erosion and deposition.
- Features such as cliffs, platforms, spits, and bars illustrate the evolutionary process of coastal landforms.
20.3 Review Questions
- How is a marine terrace related to a wave-cut platform?
- Answer: A marine terrace is related to a wave-cut platform as it is essentially an uplifted wave-cut platform. Both features are formed by marine erosion along coastlines. While a wave-cut platform is a flat, benchlike surface left behind by the receding cliff due to wave erosion, a marine terrace is a wave-cut platform that has been uplifted above sea level by tectonic forces.
- Describe the formation of each labeled feature in Figure 20.17.
- Answer:
- A: Initially irregular coast with headlands and bays.
- B: Formation of wave-cut cliffs due to erosion by pounding surf.
- C: Retreat of cliffs creates wave-cut platforms.
- D: Deposition of sediment in bays and formation of spits.
- E: River sediment fills bays while spits grow, forming baymouth bars.
- F: Final stage with a generally straight, smooth coast resulting from erosion and deposition processes.
- Answer:
- List three ways that a barrier island may form.
- Answer:
- Barrier islands may form from spits that were severed from the mainland by wave erosion or rising sea levels.
- They can also be created when turbulent waters heap up sand scoured from the ocean bottom along the line of breakers.
- Barrier islands may originate as former sand dune ridges along the shore during periods of lower sea levels, which were flooded as sea levels rose.
- Answer:
20.4 Contrasting American Coastlines
Definitions:
- Emergent Coasts: Coastal areas that experience either uplift or a drop in sea level, leading to the exposure of wave-cut cliffs and platforms above sea level.
- Submergent Coasts: Coastal areas that show signs of submergence due to rising sea levels or land subsidence, resulting in irregular coastlines and flooded river valleys known as estuaries.
Important Facts:
- Emergent Coasts:
- Examples include portions of coastal California and regions once buried beneath ice sheets.
- In California, uplift has resulted in the formation of marine terraces, such as the Palos Verdes Hills.
- Areas like the Hudson Bay region of Canada are still experiencing uplift, with rates exceeding 1 centimeter per year.
- Submergent Coasts:
- Characterized by irregular coastlines and estuaries formed by flooded river valleys.
- Examples include Chesapeake and Delaware Bays along the Atlantic coastline and the coast of Maine.
- Irregularities in submergent coasts are caused by the sea flooding lower river valleys while leaving ridges projecting as headlands.
- Erosion Problems on Atlantic and Gulf Coasts:
- Barrier islands, common along these coasts, face erosion issues due to their exposure to major storms.
- Storm waves cause movement of sand, erosion of dunes, and overwash, threatening coastal developments.
- Attempts to protect developments from erosion often lead to exacerbation of the problem.
- Erosion Problems on Pacific Coast:
- Narrow beaches backed by steep cliffs characterize much of the Pacific coast.
- Interruption of natural sand supply by dams for irrigation and flood control leads to beach narrowing.
- Narrowed beaches result in accelerated erosion of sea cliffs during storm events.
- Urbanization and development on cliffs contribute to erosion through runoff and destabilization.
- Coastal Vulnerability:
- Sporadic occurrence of storms leads to variable shoreline erosion along the Pacific coast.
- Damage from erosion is often attributed to storms rather than human interventions like coastal development and sediment-trapping dams.
- With rising sea levels, increased erosion and sea-cliff retreat are expected along many parts of the Pacific coast in the future.
2.4 Review Questions
- Are estuaries associated with submergent or emergent coasts? Explain.
- Estuaries are associated with submergent coasts. These coastal features are formed when sea levels rise or the land adjacent to the sea subsides. Estuaries occur when lower river valleys are flooded by the rising sea, leaving ridges projecting as headlands. This process characterizes submergent coasts, where the coastline shows signs of being submerged due to rising sea levels.
- What observable features would lead you to classify a coastal area as emergent?
- Observable features indicating an emergent coast include:
- Exposed wave-cut cliffs and platforms above sea level.
- Marine terraces formed by the uplift of former wave-cut platforms.
- Terraces at various elevations indicating multiple episodes of uplift.
- Ancient shorelines and coastal features high above current sea levels.
- These features suggest that the coastal area has experienced uplift or a drop in sea level, leading to the exposure of previously submerged landforms.
- Observable features indicating an emergent coast include:
- How might building a dam on a river that flows to the sea affect a coastal beach?
- Building a dam on a river that flows to the sea can have several effects on a coastal beach:
- Reduction of Sediment Supply: Dams trap sediment that would naturally flow downstream to replenish coastal beaches. This reduction in sediment supply can lead to beach erosion and narrowing.
- Altered River Mouth Dynamics: Dams can modify the natural flow of rivers, impacting sediment deposition at river mouths. Reduced sediment transport to the coast can result in diminished sand deposition on beaches, affecting their stability.
- Increased Coastal Vulnerability: With reduced sediment supply, coastal beaches may become more vulnerable to erosion from wave action and storm events. Narrowed beaches offer less protection to coastal infrastructure and can lead to increased property damage during storms.
- Overall, building a dam on a river can disrupt the natural processes that maintain coastal beaches, resulting in erosion and loss of coastal habitat.
- Building a dam on a river that flows to the sea can have several effects on a coastal beach:
20.5 p.580 Track Forecasts
Study Guide: Track Forecasts
1. Definition:
- Track Forecast: The predicted path of a hurricane or tropical cyclone. It is crucial for anticipating the trajectory of the storm and making informed decisions regarding evacuation and preparedness measures.
2. Importance:
- Critical Information: Track forecasts provide essential information for assessing the potential impact of a hurricane on specific regions, allowing authorities to issue timely warnings and evacuation orders.
- Evacuation Planning: Accurate track forecasts enable the identification of areas at risk of storm surge and other hazards, facilitating efficient evacuation planning and minimizing loss of life.
- Improving Accuracy: Advances in technology and forecasting techniques have led to significant improvements in track forecast accuracy over the years, enhancing preparedness and response efforts.
3. Historical Trends:
- Improving Accuracy: Over the past two decades, track forecast accuracy has steadily improved. Current 5-day track forecasts are as accurate as the 3-day forecasts from 20 years ago, indicating substantial progress in hurricane prediction capabilities.
- Forecast Uncertainty: Despite improvements, forecast uncertainty still necessitates issuing hurricane warnings for relatively large coastal areas. Approximately one-quarter of the warning area typically experiences hurricane conditions.
4. Forecast Cone:
- Definition: When the National Hurricane Center issues a hurricane track forecast, it is represented as a "forecast cone."
- Probable Track: The cone depicts the probable path of the storm's center, enclosing the area swept out by a series of circles along the forecast track (e.g., at 12 hours, 24 hours, 36 hours, etc.).
- Prediction Confidence: The circles within the cone expand as the forecast extends into the future, indicating increasing uncertainty. The entire track of the storm is expected to remain within the cone approximately 60 to 70 percent of the time.
5. Practical Implications:
- Public Awareness: Understanding the forecast cone and its implications helps the public and emergency responders prepare for potential hurricane impacts, including storm surge, high winds, and heavy rainfall.
- Emergency Management: Emergency management agencies use track forecasts to allocate resources, implement evacuation plans, and coordinate response efforts, enhancing community resilience and disaster preparedness.
6. Ongoing Advancements:
- Technological Innovation: Ongoing advancements in satellite imagery, computer modeling, and data assimilation techniques continue to enhance the accuracy and reliability of hurricane track forecasts.
- Collaborative Efforts: International collaboration among meteorological agencies and research institutions contributes to the development of more sophisticated forecasting models and tools, further improving track forecast accuracy.
Key Takeaway:
Accurate track forecasts are essential for informing evacuation decisions, mitigating risks, and reducing the impact of hurricanes on coastal communities. While significant progress has been made in forecast accuracy, ongoing efforts are needed to further enhance predictive capabilities and ensure effective disaster preparedness and response.
20.6 Stabilizing the Shore
1. Overview:
- Shoreline Erosion: Shoreline erosion is a dynamic process that poses significant challenges to coastal communities. Various methods are employed to address erosion problems and protect coastal property.
2. Hard Stabilization:
- Definition: Hard stabilization involves constructing structures to protect the coast from erosion and prevent sand movement.
- Examples: Jetties, groins, breakwaters, and seawalls are common forms of hard stabilization.
- Drawbacks: Hard stabilization can disrupt natural processes, cause unintended consequences such as beach erosion downstream, and require ongoing maintenance.
3. Jetties:
- Purpose: Jetties are built at the entrances of rivers and harbors to prevent sediment deposition in navigation channels.
- Impact: Jetties can impede natural sand movement, leading to beach erosion on one side while causing sediment buildup on the other.
4. Groins:
- Function: Groins are barriers constructed perpendicular to the shoreline to trap sand moving parallel to the coast.
- Issues: Groins can disrupt natural sediment transport, leading to erosion downstream and the proliferation of groin fields.
5. Breakwaters and Seawalls:
- Description: Breakwaters protect boats by creating a quiet water zone near the shoreline, while seawalls armor the coast against wave action.
- Consequences: Breakwaters can disrupt sand movement and lead to beach filling, while seawalls accelerate erosion by reflecting wave energy seaward.
6. Alternatives to Hard Stabilization:
- Beach Nourishment: Involves adding sand to beaches to widen the shoreline and mitigate erosion, but it is costly and temporary.
- Changing Land Use: Involves relocating or abandoning vulnerable structures in high-hazard coastal areas to allow natural beach processes to occur.
7. Practical Examples:
- New York's Staten Island: After Hurricane Sandy, vulnerable shoreline areas were transformed into waterfront parks to protect inland properties and provide recreational space.
- Policy Shift: Some coastal communities are reconsidering land-use policies to prioritize safety and resilience over coastal development.
8. Considerations:
- Cost vs. Benefit: Evaluating the effectiveness and sustainability of shoreline stabilization methods requires consideration of costs, environmental impacts, and long-term benefits.
- Community Engagement: Coastal management decisions should involve input from stakeholders, policymakers, scientists, and engineers to balance competing interests and ensure sustainable coastal development.
Key Takeaway:
Addressing shoreline erosion requires a multifaceted approach that considers both natural processes and human activities. While hard stabilization techniques offer immediate protection, alternatives such as beach nourishment and changing land use policies prioritize long-term resilience and environmental sustainability. Coastal management strategies should strive for a balance between protecting property and preserving natural coastal ecosystems.
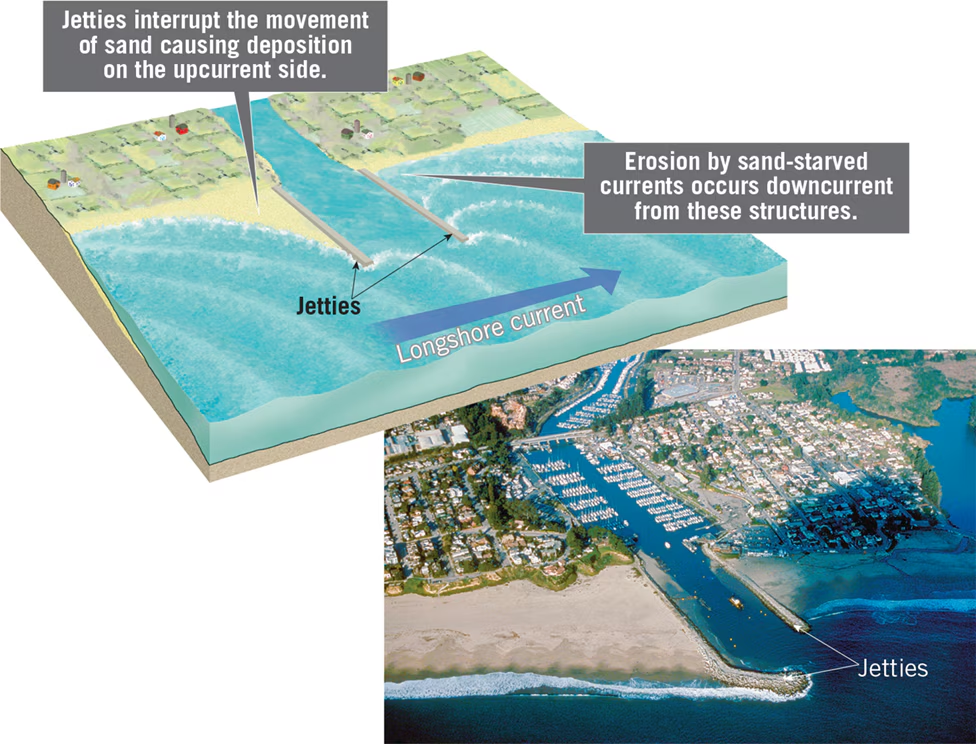
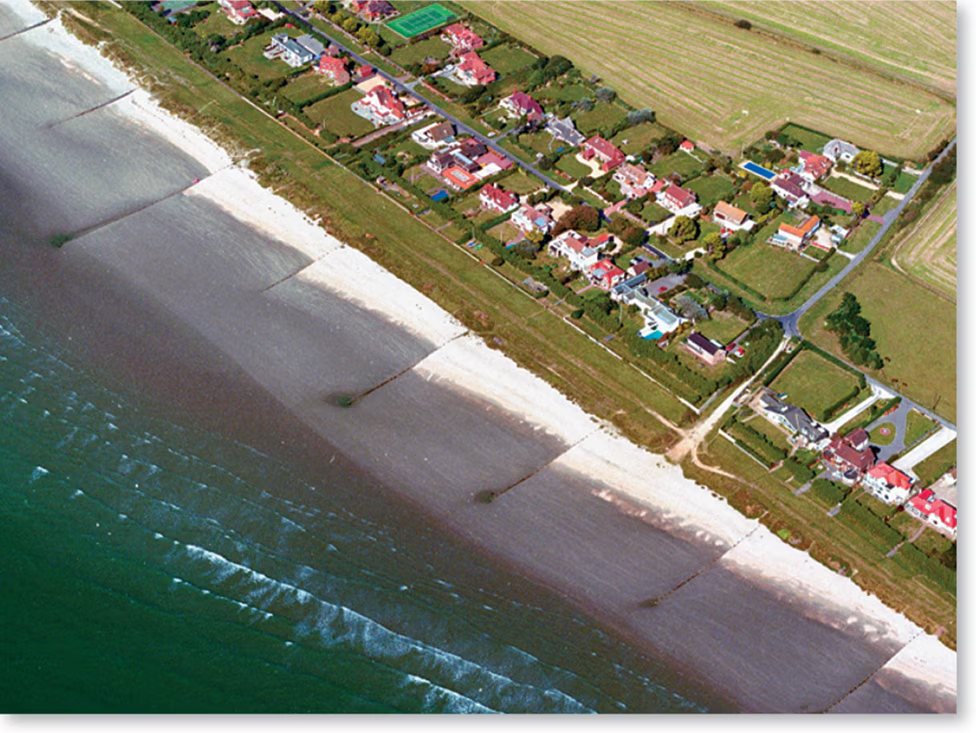
Groins
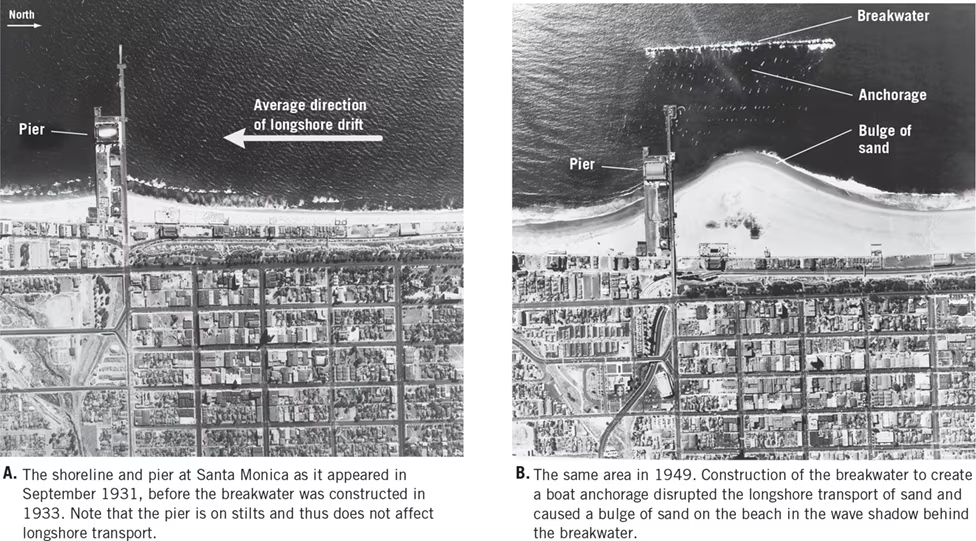
20.6 Review Questions
1. List at least three examples of hard stabilization and describe what each is intended to do:
a) Jetties:
- Intended Purpose: Jetties are built at the entrances of rivers and harbors to prevent sediment deposition in navigation channels.
- Function: They help maintain navigation channels by confining water flow to a narrow zone, preventing sediment accumulation and ensuring safe passage for ships.
- Impact on Sand Distribution: Jetties can disrupt natural sand movement, causing erosion on one side where sediment accumulates and deposition on the other side where sediment is trapped.
b) Groins:
- Intended Purpose: Groins are barriers constructed perpendicular to the shoreline to trap sand moving parallel to the coast.
- Function: They are designed to retain sediment and widen beaches by interrupting the longshore drift of sand along the coastline.
- Impact on Sand Distribution: Groins can disrupt natural sediment transport, leading to erosion downstream of the structure and the formation of groin fields, where sediment accumulates updrift.
c) Seawalls:
- Intended Purpose: Seawalls are vertical or sloping structures built parallel to the shoreline to armor the coast against wave action.
- Function: They protect coastal property from erosion by reflecting wave energy seaward and reducing the impact of waves on the shore.
- Impact on Sand Distribution: Seawalls can accelerate erosion by preventing natural sand replenishment from the sea, leading to the narrowing or disappearance of beaches in front of the wall.
2. What are two alternatives to hard stabilization, and what are the potential problems associated with each?
a) Beach Nourishment:
- Alternative Method: Beach nourishment involves adding sand to beaches to widen the shoreline and mitigate erosion.
- Potential Problems:
- Costly: Beach nourishment projects require significant financial investment for the acquisition and transportation of sand.
- Temporary Solution: The replenished sand is subject to the same erosional forces as the original beach, requiring periodic replenishment.
- Environmental Impact: Sourcing sand from offshore areas or inland quarries can disrupt ecosystems and habitats.
b) Changing Land Use:
- Alternative Method: Changing land use involves relocating or abandoning vulnerable structures in high-hazard coastal areas to allow natural beach processes to occur.
- Potential Problems:
- Social Resistance: Residents and property owners may resist relocating or abandoning coastal properties due to sentimental attachment or financial interests.
- Economic Impacts: Relocating infrastructure and properties away from the coast can result in economic losses for individuals and communities reliant on coastal tourism and development.
- Governance Challenges: Implementing land-use policies that prioritize safety and resilience over coastal development requires coordination among various stakeholders and regulatory bodies.
20.7 Tides
1. Causes of Tides:
- Tides are the daily changes in the elevation of the ocean surface caused by the gravitational interactions of Earth with the Moon and Sun.
- Sir Isaac Newton explained that ocean tides result from the gravitational attraction exerted upon Earth by the Moon and, to a lesser extent, by the Sun.
- The gravitational force of the Moon causes two tidal bulges on Earth's surface, one on the side facing the Moon and the other on the opposite side.
2. Monthly Tidal Cycle:
- The primary influence on tides is the Moon, which makes one complete revolution around Earth every 29.5 days.
- Near the times of new and full moons, the combined gravitational forces of the Moon and Sun cause larger tidal bulges, resulting in spring tides.
- At first and third quarters of the Moon, the gravitational forces of the Moon and Sun act at right angles, resulting in smaller tidal ranges known as neap tides.
- Each month experiences two spring tides and two neap tides, occurring approximately one week apart.
3. Tidal Patterns:
- Tidal patterns are influenced by various factors, including the shape of the coastline, ocean basin configuration, and water depth.
Three main tidal patterns exist worldwide:
a) Diurnal Tides: Characterized by one high tide and one low tide each tidal day.
b) Semidiurnal Tides: Exhibit two high tides and two low tides of approximately equal heights each tidal day.
- c) Mixed Tides: Similar to semidiurnal tides but with a large inequality in high and low water heights, occurring each tidal day.
4. Tidal Currents:
- Tidal currents are horizontal flows of water that accompany the rise and fall of the tide, induced by tidal forces.
- Flood currents move into coastal zones as the tide rises, while ebb currents move seaward as the tide falls.
- Slack water refers to periods of little or no current between flood and ebb.
- Tidal currents can be rapid in narrow areas such as bays, estuaries, and straits, where they may attain high speeds.
- Tidal currents are not generally major agents of erosion and sediment transport, but they can create deposits called tidal deltas in certain areas, particularly around inlets.
Key Concepts:
- Tides result from gravitational interactions between Earth, the Moon, and the Sun.
- Monthly tidal cycles include spring tides (large tidal ranges) and neap tides (small tidal ranges).
- Tidal patterns vary globally, with diurnal, semidiurnal, and mixed tides.
- Tidal currents accompany the rise and fall of tides and can influence coastal dynamics, including erosion and sediment transport.
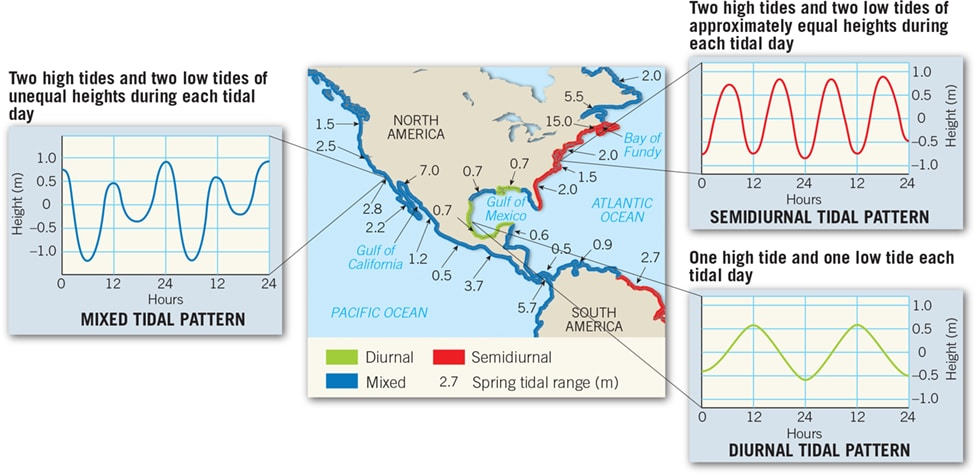
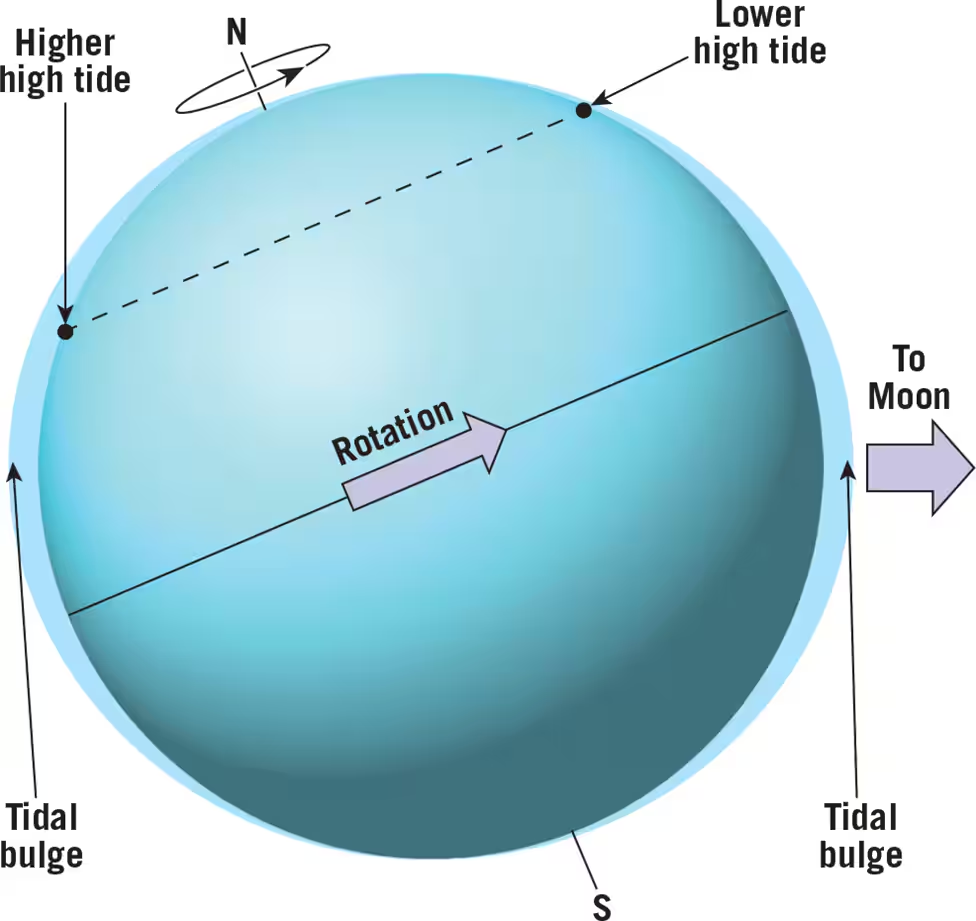
If Earth were covered to a uniform depth with water, there would be two tidal bulges: one on the side of Earth facing the Moon (right) and the other on the opposite side of Earth (left). Depending on the Moon’s position, tidal bulges may be inclined relative to Earth’s equator. In this situation, Earth’s rotation causes an observer to experience two unequal high tides during a day.
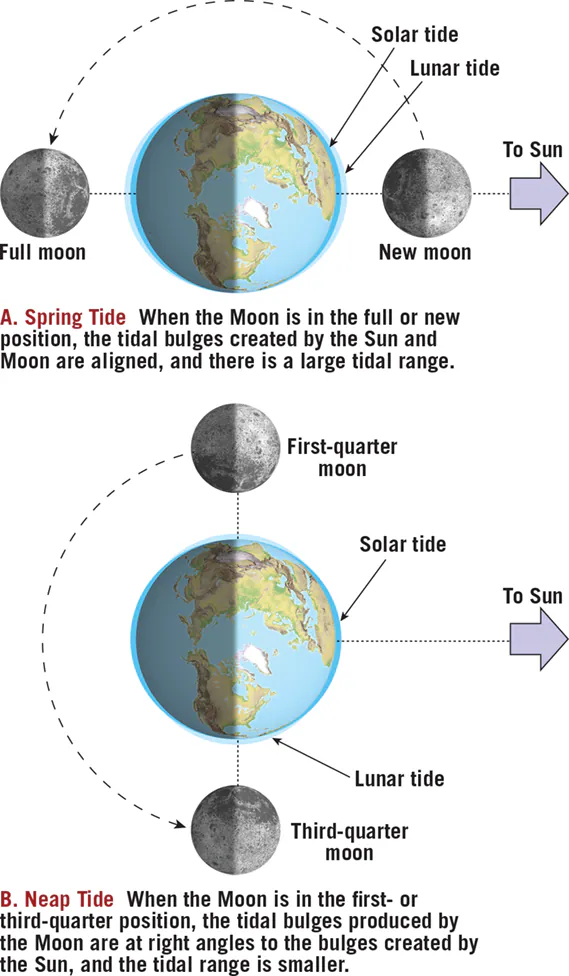
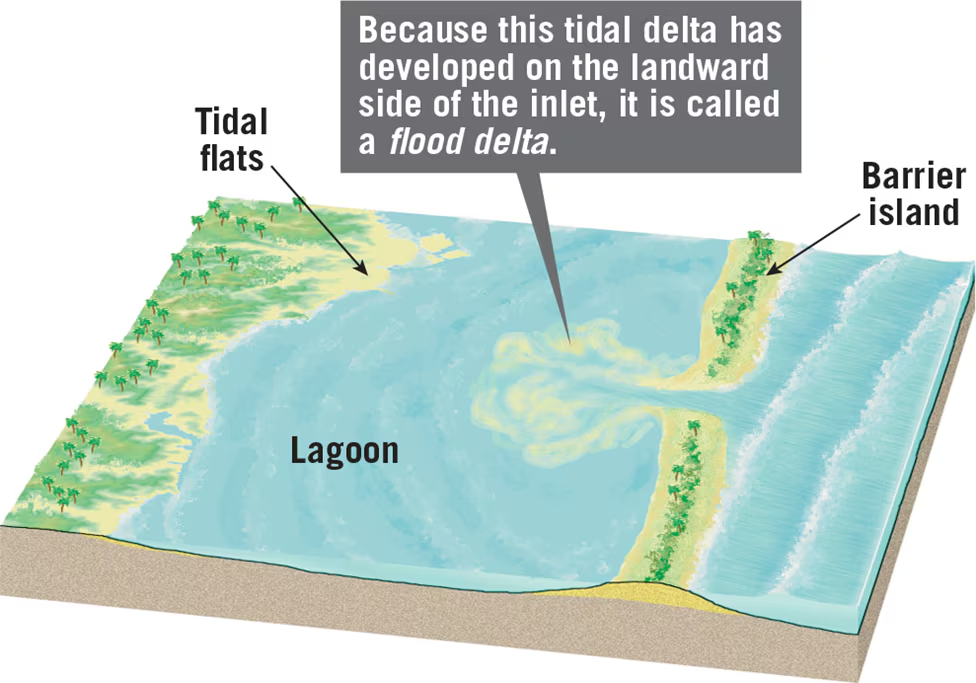
20.7 Question Review
Explain why an observer can experience two unequal high tides during a single day:
- An observer can experience two unequal high tides during a single day due to the inclination of the tidal bulges relative to Earth's equator. Depending on the Moon's position, the tidal bulges may be inclined, resulting in variations in tidal heights. When the tidal bulge is inclined, the gravitational forces acting on the water are not balanced, leading to unequal high tides. As Earth rotates, an observer may pass through areas where the tidal bulge is more pronounced, resulting in a higher high tide, and areas where it is less pronounced, leading to a lower high tide. Therefore, the observer experiences two unequal high tides within a single day.
- Distinguish between neap tides and ebb tides:
- Neap tides occur when the gravitational forces of the Moon and Sun act on Earth at right angles, partially offsetting each other's influence. As a result, the tidal range is smaller during neap tides compared to spring tides. Neap tides occur approximately during the first and third quarters of the Moon.
- Ebb tides, on the other hand, refer to the period of tidal current flow when seaward-moving water generates ebb currents. These currents occur as the tide falls and the water moves away from the coast. Ebb tides are a specific phase of the tidal cycle and are characterized by the movement of water from coastal areas towards the open ocean.
- Contrast flood current and ebb current:
- Flood current refers to the period of tidal current flow when water moves into coastal zones as the tide rises. This movement of water towards the coast is driven by the rising tide and is known as flood current. Flood currents typically occur during the first half of the tidal cycle.
- Ebb current, on the other hand, occurs during the period of tidal current flow when water moves seaward as the tide falls. As the tide ebbs, water flows away from the coast towards the open ocean, generating ebb currents. Ebb currents are characteristic of the second half of the tidal cycle and represent the outward movement of water from coastal areas.
Practice Questions:
3.) Geologists have discovered long, generally straight chains of volcanic islands within ocean basins that get progressively younger or progressively older depending on the direction of travel along the volcanic chain. The Hawaiian Islands are an example of such a chain. What is the origin of such a volcanic chain?
a.) magma intruding along the boundary between two lithospheric plates.
b.) magma intruding from a large region of flood basalts.
c.) magma intruding within an island arc.
d.) magma intruding along a mid-ocean fracture zone.
e.) magma intruding from a hotspot within the mantle.
39.) Suppose that you discovered coal deposits over glacial tills in rocks at the northern tip of Greenland. How would you interpret this discovery.
a.) The earth's cool conditions once favored rapid planet growth at the latitude of northern Greenland.
b.) You conclude that you made a mistake in identifying the till because coal can not possibly be deposited on top of glacial tills.
c.) The continent of Greenland must have drifted from a polar latitude to a tropical latitude and back again.
d.) The coal was deposited by the present continental glaciers covering the Greenland ice cap.
e.) You make a note of this outcrop but you are not excited because coal and till are commonly found in the same outcrop.
46.) Recall the block diagrams of faults in your text book. What is the name of the fault which has a hanging wall moving downward relative to the foot wall?
a.) a reverse fault.
b.) a normal fault.
c.) a strike-slip fault.
d.) a transform fault.
e.) a thrust fault.
55.) The following two locations are examples of hot spots:
a.) State College, PA and Washington D. C.
b.) Hawaii and the East Pacific Rise.
c.) Hawaii and Yellowstone.
d.) Mid Atlantic Ridge and Cascades.
e.) Sumatra and Hawaii.
64.) Which of the following geological periods is oldest?
a.) Cretaceous.
b.) Tertiary.
c.) Jurassic
d.) Cambrian.
e.) Devonian.
98.) Of the three types of seismic waves, which cause the most damage during an earthquake?
a.) compressional waves.
b.) shear waves.
c.) transverse waves.
d.) longitudinal waves.
e.) surface waves.